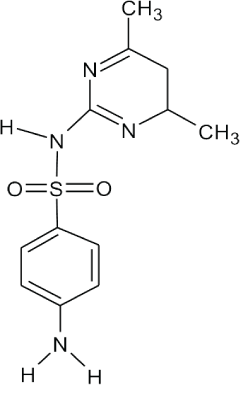
Figure 1: Structure of Sulfamethazine (SMZ).
Andreas Fath1* Annika Over2 Veronika Wank2 JE McCaskie3
1Department of Medical and Life Sciences, Furtwangen University, Germany*Corresponding author: Andreas Fath, Department of Medical and Life Sciences, Furtwangen University, Germany, E-mail: fath@hs-furtwangen.de
Many micropollutants such as, excreted or improperly discarded pharmaceuticals including antibiotics, are resistant to conventional biological treatments of waste waters. Non-conventional techniques have been designed to eliminate these substances in modified sewage treatment facilities and also at liquid manure treatment sites at livestock farms. Antibiotics such as, Sulfamethazine (SMZ) and Tetracycline (TC) are two different classes of anti-infection agents used in large amounts in human and veterinary medicine and are used as chemical models in this work. These antibiotics were electrochemically oxidized in cattle urine and water media at the lab scale of 300 milliliter-reactor using lead dioxide (Pb/PbO2 ) electrodes and efficiency results were analyzed by HPLC-MS/MS and by UV-spectroscopy.
The method of electrochemical decomposition of antibiotics in water and cattle urine was 95% efficient in the concentration range 0.50-10.00 mg/l (0.5 ppm-10 ppm). Specifically, at current density of 30 mA/cm2 >95% degradation of 10 ppm sulfamethazine was achieved in cattle urine and 90% in water within 60 min. Tetracycline in water was >95% decomposed in 40 min and to the same degree within 60 min in cattle urine.
Electrochemical decomposition; Antibiotics; Wastewater treatment; Cattle urine; Tetracycline (TC); Sulfamethazine (SMZ); Sulfadimidine (SDM); Micropollutants
Antibiotics are used in large quantities in human and veterinary medicine for therapeutic and prophylactic reasons, but a large amount of the drugs administered is excreted in unchanged form into the environment, especially from large scale livestock farming sources.
The accumulation of these micropollutants in water and plants provides a path into human food chain and can promote the development of drug resistant bacteria. To reduce the environmental impact, industrial and agricultural wastewater should be treated to remove pollutants at its source [1-3].
The micropollutants are resistant to conventional biological treatments of waste waters. Non-conventional techniques, such as electrochemical decomposition, have been designed to eliminate these substances in modified sewage treatment facilities and also at liquid manure treatment sites at livestock farms.
Electrochemical decomposition of organic compounds, such as dyes or complex surfactants, to simpler intermediates or ultimately to water and carbon dioxide is a known reaction [4]. Electrochemical degradation processes are characterized by their robustness and high efficiency and can compete with most advanced oxidation technologies, such as ozonation. Fath, et al. has shown that substances such as Perfluorinated Alkyl Substances (PFAs) and hexavalent chromium from industrial processes are effectively decomposed by electrochemical oxidation [5]. Electrode material costs and durability has inhibited wide use of electrochemical decomposition of trace materials in waste to cases where other technologies fail to provide efficient performance [5,6].
Practical uses of electrochemical processes require dimensionally stable electrode materials. For example, electrodes with Borondoped Diamond films (BDD electrodes) are frequently studied due to their stability and hydroxyl radical generation [7]. On the other hand, BDD electrodes are very costly and difficult to manufacture on a large scale. Recent studies have shown utility for BDD and also Ti/ IrO2 anodes in oxidizing trace contaminants, including antibiotics in wastewater [8]. Often, mixed electrodes, e.g. platinized titanium are used. But platinized electrodes are also expensive and less inert than required. As a result, solvated platinum (Pt+2) can cause unwanted chemical reactions [9]. By using simple to manufacture, low cost electrode materials, such as Pb/PbO2 -electrodes, this method is economical and adaptable for different applications like industrial process water treatment or on-site treatment of sewage. Composite electrodes, e.g. lead dioxide coated titanium Ti/PbO2 , are used as an anode. Lead dioxide electrodes are reasonable in cost with high conductivity, and are chemically, mechanically and electrochemically stable. Recent studies in high surface area PbO2 anodes have proven their utility in efficient degradation of metalaxyl and 2,4 D herbicide and other organic compounds in waste water [10-12]. In addition, Martinez reports extensive degradation studies of pollutants by electrochemistry including PbO2 and BDD and the chemical identity of products formed [13].
In addition, lead dioxide electrodes exhibit high oxygen overpotential for degrading materials in wastewater. The applied current density avoids lead release from the transpassive lead anode into the solution, so that the concern of secondary pollution of soluble ionic lead species can be excluded. Sulfamethazine (SMZ) and Tetracycline (TC), two different classes of antibiotics used in large amounts in human and veterinary medicine are used as chemical models in this work. Previous work has shown that Pt/Ti electrodes exhibited no significant difference in the rate of decomposition compared with Pb/PbO2 electrodes, neither in the PFAs case, nor with the decomposition of the selected antibiotics in cattle urine [6].
The current work aims to test and compare the electrochemical degradation of SMZ, figure 1 and TC figure 2, in water and cattle urine with low cost Pb/PbO2 -electrodes.
Electrochemical degradation of another sulfonamide antibiotic, Sulfamethoxazole (SMX) has been studied [14]. Due to its high solubility, SMZ is regarded as a mobile veterinary medicine, with a high risk of dissolving in surface waters or leaching into groundwater. Its solubility behavior depends on the soil matrix, the irrigation quantity, the soil grain size distribution, pH, the organic content, and the soil moisture.
Figure 1: Structure of Sulfamethazine (SMZ).
Figure 2: Structure of Tetracycline (TC).
Tetracyclines (TCs) are counted among the clinically most important antimicrobial pharmaceuticals due to their wide range of action and are therefore frequently used in veterinary and human medicine [1]. The stability of tetracyclines in aqueous solutions varies according to pH, temperature and derivative [15,16].
Electrochemical decomposition of the antibiotics in water and cattle urine was carried out in a single-compartment electrochemical cell working in batch operation mode. The cell anode was prepared Pb/PbO2 -foil and cathode was stainless steel (AISI 304). The active surface area of both electrodes was each 120 cm2 and an electrode gap was held to 7 mm. In each experiment, 270 ml of 10 ppm tetracycline and sulfamethazine dissolved in deionized water or cattle urine was electrochemically decomposed. In order to increase the conductivity of the aqueous solution, sulfuric acid was additionally added to pH 2. On the other hand, nothing was added to the urine test sample due to its existing high conductivity of 31.2 mS. The voltage supply source, was rectifier TG 411 by Degussa. The current density was set to 30 mA/ cm2 . At different time intervals, aliquots of 2 ml of test solution were analyzed by UV-spectroscopy and HPLC-MS/MS.
For the electrochemical decomposition experiments a planar anode with an active surface of about 120 cm2 was prepared according to Yahiaoui et al. [17,18].
UV-spectroscopic measurements of sulfamethazine (SMZ, 99%, CAS Number 57-68-1, Sigma-Aldrich): The degradation of SMZ was measured with a colorimetric test, Bratton- Marshall method, a quantitative technique for analysis of sulfonamides. Following the protocol of the University of Jena, the quantification of the “BrattonMarshall reagent” is carried out in a modified form following standard procedure [19,20].
The sample preparations are measured at 540 nm in a UV/VIS spectrophotometer Lambda XLS by Perkin Elmer. The quantification is carried out by comparison with a calibration curve for SMZ in concentration range of 100 to 0.4 mg /L.
UV-spectroscopic measurements of tetracycline: (TC, CAS Number 60-54-8, Alfa Aesar) The epimers and derivatives of tetracycline have two characteristic absorption UV spectra which can be quantified. Depending on the pH value the BCD chromophore absorbs light in the wavelength range between approx. 360 and 380 nm while the A-chromophore absorbs light in the wavelength range between 260 and 280 nm [15,16]. The UV instrument was zeroed on a sample in dilute sulfuric acid to adapt the pH value to the degradation sample pH. Tetracycline concentrations of 10 to 0.5 ppm in deionized water with sulfuric acid were used as calibration standards.
Sample preparation by Solid-Phase Extraction (SPE) and HPLC-MS/MS analysis: Samples of the degradation experiments in water with an initial sulfamethazine and tetracycline concentration of 10 ppm were prepared by a solid-phase extraction (SPE) to remove sulfates and other contaminants according to Gajda and Posyniak [21]. The analytes were then eluted by methanol including 1 ppm sulfamethoxazole (SMX in methanol) as internal standard and analyzed by HPLC-MS/MS by Eurofins WEJ Contaminants GmbH (Hamburg, Germany).
The antibiotic mixture of SMZ and TC was electrochemically decomposed at a current density of 30 mA/cm², then pre-purified by solid-phase extraction and subsequently analyzed by HPLC-MS/MS. The following table 1 indicates the conditions in the reactor, such as the average voltage V, the current I, current density CD, the temperature T, as well as other degradation values. The concentration is given in percent for the HPLC-MS/MS method, as well as for the colorimetry C, at 5 and 30-minutes degradation time.
pH-value | Temperature in °C | Volt | I Amp | CD mA/cm2 | % Concentration at 5 min | % Concentration at 30 min |
2 | 45 | 9.6 | 3 | 25 | HPLC: 45 C: 78 |
HPLC: 3 C: 25 |
Table 1: Parameters and results of the electrochemical degradation of SMZ.
Figure 3 shows results of the degradation samples by HPLC analysis and the colorimetric method. Furthermore, the SMZ recovery rate was between 90 and 106%. Both detection methods showed similar trends, with the HPLC method detecting a higher degradation level of SMZ than colorimetry on average. At 60 min, the curves are aligned. As the average recovery rate of SMZ in the SPE is 98%, it can be assumed that the HPLC method is more accurate. In the colorimetric method, the aromatic amines of the SMZ are used as coupling partners for the formation of the diazonium salt and thus a slightly lower decomposition is achieved compared to the HPLC method. Presumably degradation products of the SMZ contain aromatic amines which are also converted into a diazonium salt and interfere with accuracy of the colorimetric method. A quantification of the SMZ samples by the colorimetric method is nevertheless suitable, since the percent deviation is minor and the final results are comparable.
Figure 3: Concentration of SMZ and comparison of the analytical methods HPLC-MS / MS with the colorimetric quantification.
Aqueous test solution (270 ml) adjusted to pH 2, containing 10 ppm TC and 10 ppm SMZ, was electrochemically degraded at about 25 mA/ cm2, respectively. The degradation samples were analyzed as described via HPLC-MS/MS and UV-spectroscopy. HPLC-MS/MS analysis has a detection limit of TC and SMZ of 0.025 ppm. The high recovery rate of 90 to 106% for TC and SMZ gives high confidence in the accuracy of the results by these methods. As a control the more sensitive specific HPLC method, TC and SMZ could be quantified independently of each other and the results from each method could be compared.
The electrochemical decomposition of the A and BCD tetracycline chromophores, as well as the degradation rates determined via HPLC MS/MS are shown in figure 4 (solid lines represent best fit to data points). In figure 4, the A chromophore is generally decomposed more slowly than the BCD chromophore. This can be attributed to the tetracycline structure because the BCD-chromophore with its three-ring structure is less stable than the simple A-chromophore ring. The spectroscopic measurements of the BCD chromophore are comparable to the tetracycline quantification by HPLC-MS/MS analysis with deviation values of 5-15%. Thus, the spectroscopic measurement of the BCD chromophore offers a faster, more convenient quantification route than the HPLC method in TC concentration ranges >0.5 ppm
Figure 4: Comparison of the UV-spectroscopic and HPLC-MS/MS-analysis of TC electrochemical decomposition in pH 2 water.
Degradation experiments of TC (10 ppm) in aqueous solution at pH 2 at 10, 30 and 40 mA/cm2 current densities are shown in figure 5. In each case, 270 ml of 10 ppm tetracycline solution pH 2 were electrochemically degraded for 45 min and 2 ml sample aliquots were taken at time intervals.
Figure 5 shows that electrochemical elimination accelerates with increasing current density, as previously shown for sulfamethoxazole by Lin H, et al. [14]. However, since no significant difference in rate is observed at 40 mA/cm2 compared with 30 mA/cm2, subsequent experiments were conducted at 30 mA/cm2.
Figure 5: Decomposition of tetracycline in pH 2 water at different current densities.
In these experiments, 270 ml aqueous solution pH 2 and 270- ml cattle urine spiked with 10 ppm tetracycline TC and SMZ were decomposed at 30 mA/cm2. The corresponding decomposition curves are shown in figure 6 and figure 7.
Figure 6: Comparison of the electrochemical decomposition of tetracycline in water (black line) and cattle urine (blue line).
Figure 7: Comparison of the electrochemical decomposition SMZ in water (red line) and cattle urine (black line).
As shown in figure 6, >95% tetracycline (10 ppm) in water was decomposed in 40 minutes degradation time and >95% in 60 minutes in cattle urine. Figure 7 shows the degradation of sulfamethazine (10 ppm) after 60 min in water at >97% and in cattle urine at 87%. The two antibiotics were thus successfully decomposed in wastewater (cattle urine) and water by electrochemical processes. To date, no electrochemical studies of elimination of antibiotics in simulated wastewater, such as cattle urine, has been studied. The figures show that both substances can be degraded slightly faster in water than in more complex matrices, such as urine. However, cattle urine would be a normal waste stream which could be readily treated with this advanced oxidation process than pure water matrix. No acid addition is required to increase the conductivity of the urine in order to effectively decompose the micropollutants. However, to eliminate foaming during processing of urine an addition of defoamer may be studied in the future scale up work.
PbO2 anode can react with water to form hydroxyl radicals which themselves can react with the organic species to form unstable intermediates and ultimately lead to CO2 and water [22].
PbO2 + H2O → PbO2 [OH. ] + H+ + e-
PbO2 [OH. ]+ R (e.g. SMZ or TC) CO2 +H++e- +intermediates (intermediates such as, polymers or carboxylic acids, NOx compounds, quinones, azides)
An electrochemical treatment process of liquid manure (cattle urine) is suitable to prevent dissolved antibiotic release into groundwater. Additionally, a rapid, low cost photometric test is described to monitor progress of treatment process.
Funded by the Integro GmbH working group “Clear Water”.
Download Provisional PDF Here
Article Type: RESEARCH ARTICLE
Citation: Fath A, Over A, Wank V, McCaskie JE (2018) Electrochemical Decomposition of Sulfamethazine and Tetracycline in Water and Cattle Urine. Int J Water Wastewater Treat 4(2): dx.doi.org/10.16966/2381-5299.155
Copyright: © 2018 Fath A, et al. This is an open-access article distributed under the terms of the Creative Commons Attribution License, which permits unrestricted use, distribution, and reproduction in any medium, provided the original author and source are credited.
Publication history:
All Sci Forschen Journals are Open Access