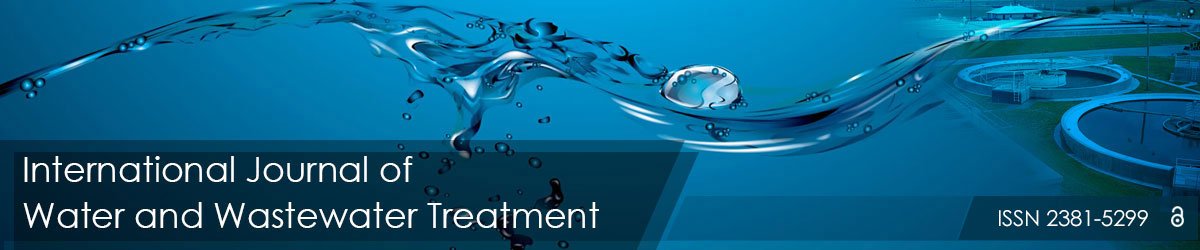
Full Text
Lindsey Goodwin Irene Carra Pablo Campo Ana Soares*
Cranfield Water Science Institute, Cranfield University, Cranfield, MK43 0AL, UK*Corresponding author: Ana Soares, Cranfield Water Science Institute, Cranfield University, Vincent Building, Cranfield, Bedfordshire, MK43 0AL, UK, Tel: +44 (0) 1234 758121; E-mail: a.soares@cranfield.ac.uk
The pesticide production industry generates a high strength wastewater containing a range of toxic pollutants (2,4-dichlorphenoxy acetic acid: 2,4-D; 4-(2,4-dichlorphenox) propionic acid: 2,4-DP; 4-(2,4-dichlorophenox) butyric acid: 2,4-DB; 2,4-dichlorophenol: 2,4-DCP; 2,4,6-trichlorophenol: 2,4,6-TCP; 4-chlororthocresol: PCOC; 4-chloro-2-methyl phenoxyacetic acid: MCPA, 4-(4-chloro-2-methylphenoxy) butyric acid: MCPB and 2-(4-chloro-2-methylphenoxy) propionic acid: MCPP). These pesticides can enter the natural environment and water sources if not removed in a wastewater treatment plant. Treated effluents are regulated by legislation such as the Water Framework Directive (WFD). Most studies found in literature focused on synthetic solutions, synthetic wastewater, at lab-scale or pilot-scale. Although these studies can provide information on the removal mechanisms and provide a comparison between process efficiency, they have limited practical applicability. The process that has been more widely used to treat high strength wastewaters rich in recalcitrant compounds at full-scale, is the combination of biological/granular activated carbon and granular activated carbon/biological processes. The pesticide production wastewater contains a variety of compounds, that can be removed by 80-90% using biological processes (such as membrane bioreactors) and granular activated carbon has been shown to selectively remove the pesticides, potentially creating a high quality effluent. Nevertheless, in order to assert processes design, efficiencies or costs, it is crucial to evaluate these processes experimentally.
According to the European Commission (EC) a pesticide is any substance or a mixture of substances that are intentionally used for the purpose of destroying, repelling, preventing or migrating any pest; used as a plant regulator, desiccant or defoliant or used as a nitrogen stabiliser (EC, 2016). Pesticides provide a way for regulating organisms that compete with humans for food, vitamins and minerals and prevent damage to crops, livestock and humans. In today’s society pesticides have a major role in the economic production of farming and contribute to a large-scale success in the agricultural industry worldwide.
Pesticide production takes place in centralised factories involving the synthesis and combination of large volumes of man-made chemicals. During the pesticide production process waste products are formed giving origin to wastewater that needs further treatment before it is released to the environment. The characteristic of the pesticide production wastewater varies from company to company depending on the target crop and scale of applications such as domestic to industrial. For example, in the agricultural industry the chemicals are likely to be stronger and more hazardous when compared to domestic application such as a small garden. Table 1 shows typical characteristics of pesticide production wastewater. From existing studies carried, it can be seen that there is great variability for example, pH has been reported to be extremely acidic at 0.5 [1] to extremely alkaline pH 14 [2], chemical oxygen demand (COD) and biochemical oxygen demand (BOD) have been reported in the range of 150-33750 mg/L and 30-11590 mg/L, respectively (Table 1). The key pollutants in the pesticide production wastewater include: 2,4, dichlorphenoxy acetic acid (2,4-D); 4-(2,4 dichlorphenox) propionic acid (2,4-DP); 4-(2,4, dichlorophenox) butyric acid (2,4-DB); 2,4 dichlorophenol (2,4-DCP); 2,4,6-trichlorophenol (2,4,6-TCP); 4-chlororthocresol (PCOC); 4-chloro2-methyl phenoxyacetic acid (MCPA), 4-(4-chloro-2-methylphenoxy) butyric acid (MCPB) and mecoprop (MCPP) in the range of mg/L. These pesticides greatly vary in concentrations between each production sites. Concentrations of the above pesticides have been reported up to 2500 mg/L. In the UK, the pesticides present in the wastewater are highly variable with concentrations ranging from 0.1-107 mg/L.
pH | BOD (mg/L) |
COD (mg/L) |
Total suspended solids | Ammonium (mg/L) | Phosphate (mg/L) | COD/BOD | References |
0.5-2 | 260 | 3680 | 1750 | - | 250 | 14.2 | [1] |
6-9 | 30 | 150 | 10 | - | - | 5 | [5] |
4-5 | 750-1200 | 2500-5000 | 40-50 | 40-50 | 300-400 | 3.33-416 | Jin et al.[6] |
1.5-2.5 | 6100 | 33700 | - | 3080 | 2040 | 5.52 | Cheng et al. [7] |
2-8.5 | 37.2-49.5 | 124-366 | - | - | - | 3.33-7.39 | [8] |
12-14 | 2000-3000 | 6000-7000 | 250-300 | - | - | 2.33 | Misra et al. [2] |
Table 1: Characterisation of different pesticide production wastewater
This huge variations proposes a challenge when trying to treat the wastewater as these contributing parameters are key to determining whether the pesticide wastewater can be biologically treated or not and if the wastewater composition changes frequently will the treatment be effective for each batch variation. Industrial wastewater requires bespoke treatment to successfully remove the specific compounds to safe levels before being discharged to the environment. Compounds which are not as easily removed, such as pesticides, are treated to safe levels and are monitored for compliance to protect the environment. This is achieved by reducing the quantity of pollution distributed in the environment with very strict limits. For example, for fresh water sources some recalcitrant pesticides have their own specific limits. For 2,4‐ dichlorophenoxyacetic acid (2,4‐D) the limits are 0.3 µl/L (mean) and 1.3 µl/L (95 percentile) [3]. In order to regulate and maintain safe levels of pollution in water courses (streams, rivers, sewers) several organisations in the UK monitor the water quality, these include the European Union organisations (through the Water Framework Directive – WFD), the Department of Food & Rural Affairs (DEFRA) and the Environment Agency (EA) [4].
The majority of research to date has been focused on pesticide degradation in mixtures of one to four different pesticides; the more common pesticides 2,4- dichlorphenoxy acetic acid (2,4-D), 2,4- dichlorophenol (2,4-DCP), 4-chloro-2-methyl phenoxyacetic acid (MCPA) and 4-chlororthocresol (PCOC) [9-13]. Studies using synthetic pesticide wastewater at laboratory scale have been shown to treat pesticide concentrations in the range of 0.9 – 20,000 µg/L [14] whilst studies using different types of wastewater with pesticides at laboratory scale have been shown to treat pesticides ranging from <0.36 µg/L up to 2500 mg/L [15,12]. The objective of this review was to establish the most suitable processes to treat pesticide production wastewaters with a mixture of pollutants.
Wastewater containing pesticides have been reported to be treated using conventional wastewater treatment processes such as coagulation, filtration, trickling filters and conventional activated sludge (AS) [12]. Conversely, these processes do not provide reliable effective treatment against pesticides. For instance, many studies have reported biological processes to be challenging when treating high strength wastewater due to pollutants showing toxicity and resistance towards the microorganisms [6,16]. The chlorinated herbicides 2,4-D, 2,4-DCP, 2,4,6-TCP, MCPA and MCPP will be challenging to remove using biological processes due to the structure, chemical groups and significant half-lives, therefore a pre/post treatment option would ensure that these chlorinated herbicides would be removed prior to been discharged [9].
According to the US Environment Protection Agency (EPA) the best available technology (BAT) for organic compound removal in water is granular activated carbon (GAC) due to being highly efficient at treating a wide range of organic compounds including pesticides [17]. Studies show when utilising GAC as a pre-treatment process the adsorption of pesticides on GAC is decreased due to high competition with other bulk organic matter for adsorption pores. This can lead to reduced lifespan and increased frequency of media regeneration which makes the process very expensive. Literature suggests using GAC as a post-treatment increases the efficiency for adsorbing pesticides since most of the bulk organic matter will be removed prior to using GAC columns [18]. Combining membrane bioreactor (MBR) and GAC to create a hybrid system would produce an even more enhanced high quality effluent, as the effluent with a lower concentration of organic matter and no suspended solids from the MBR would allow the GAC to target and remove the remaining recalcitrant pollutants [19].
The use of chemical processes offers an alternative option in removing recalcitrant pesticides in wastewater via chemical oxidation; for instance advanced oxidation processes (AOPs). Over recent decades AOPs have received significant attention for the removal of pollutants in both industrial and domestic wastewaters [8,10,11,20-23] due to the highly efficient treatments on recalcitrant wastewater. Advanced oxidation processes are able to achieve this by generating hydroxyl radicals which are non-selective and highly reactive [24,25,22] Removals between 51.4% to 100% removal have been achieved utilising various AOPs including UV/TiO2 [10], UV/H2 O2 [11] and Fenton process [26,8].
Although pesticides are produced in very large quantities worldwide, there is very little information about the production wastewater characterisation as well as reports on how to treat such a wastewater efficiently and economically. The aim of this review was understand the state of the art on the commercial available options to remove 9 pesticides (phenoxy acids and dichloro acids) from a high strength industrial wastewater from the pesticide production industry.
Chemical and physical properties of the chlorinated aromatic herbicides in pesticide production wastewater
The chlorinated aromatic herbicides present in the pesticide production wastewater have different chemical and physical characteristics meaning they have different environmental fates (Table 2). To understand and predict the most efficient processes to remove pollutants from wastewater, it is key to correlate the chemical and physical characteristics of the pollutants with their environmental fate such as solubility, molecular weight, structure octanol/water coefficient (Log Kow), sludge distribution coefficient (Kd), Henry’s coefficient (Hc) and compounds reactivity to hydroxyl radicals (KoH) (Table 3). Furthermore, these characteristics can help design suitable treatment processes using one or a combination of these: sorption, volatilisation, biodegradation and transformation or chemical conversion.
Solubility (mg/ L) | <2 poor solubility | 2-100 average solubility | >100 high solubility |
Log Kow | 0.5-2.5 Very hydrophilic & bioavailable | 2.5-4.0 Average hydrophobic | >4.0 Very hydrophobic |
Log Hc (atm/mol.m3) | <10-3 Volatilise | >10-3 Poor volatilisation | |
Log Kd | <0.7-1.5 Poor sorption to solids | 0.1-10 Average sorption to solids | 3.0-3.9 Strong sorption to solids |
Log Kow (1/M.S) | <109 Poor reactivity with hydroxyl radicals | >109 Good reactivity with hydroxyl radicals | |
radicals | radicals |
Table 2: Interpretation of the physical and chemical characteristics of pollutants
CAS Number | Compound name | IUPAC name | Chemical formula | Configuration | Molecular weight (g/ mol) | Solubility (mg/L) | Log Kow | Log Kd | Hc atm/ (mol.m3)** | (K on ) Reactivity between hydroxyl radicals (1/M.s) | |
94-75-7 | 2,4, dichlorphenoxy acetic acid | 2,4-D | C8H6 Cl 2O3 |
![]() |
221.04 | 890 | 2.61 | 2.95 | 5.81 × 10-9 | 5.1 × 109 | |
94-82-6 | 4-(2,4, dichlorophenox) butyric acid | 2,4-DB | C10 H10 Cl2 O3 |
![]() |
249.09 | 4385 | 3.53 | N/A | 2.29 × 10-9 (non -volatile with water) | N/A | |
120- 83-2 | 2,4 dichlorophenol | 2,4-DCP | C6H4 Cl2 O |
![]() |
163.00 | 4500 | 2.80 | 1.8 | 5.5 × 10-6 | 5.5 × 109 | |
120- 36-5 | 4-(2,4 dichlorphenox) propionic acid | 2,4-DP | C9 H8 Cl2 O3 |
![]() |
235.06 | 230 | 3.26 | -1.5 | 9.0 × 10-7 | 6.614 × 109 | |
88-06-2 | 2,4,6-trichlorophenol | 2,4,6-TCP | C6H2 Cl3 OH |
![]() |
197.45 | 800 | 3.38 | 2.3 × 10-7 | 6.3 × 1009 | ||
1570- 64-5 | 4-chlororthocresol/4- chloro-o-cresol/para- Chloro-ortho-cresol | PCOC/ 4CL2MPHE | C7 H7 ClO |
![]() |
142.58 | 2300 | 3.09 | 0.008 | 1.1 × 10-6 | N/A | |
94-74-6 | 4-chloro-2-methyl phenoxyacetic acid | MCPA | C9 H9 ClO3 |
![]() |
200.63 | 630 | 3.25 | 1.47 | 6.55 × 10-7 | 6.6 × 109 | |
94-81-5 | 4-(4-chloro-2- methylphenoxy) butyric acid | MCPB | C11H13 ClO3 |
![]() |
228.67 | 48 | 3.5 | 2.79 | 3.22 × 10-4 Pa m3 mol-1 | N/A | |
93-65-2 | 2-(4-chloro-2- methylphenoxy) propionic acid | MCPP | C10 H11 ClO3 |
![]() |
214.65 | 650 | 3.38 | -1.5 | 1.2 × 10-7 | N/A | |
122- 59-8 | phenoxyacetic acid | PAA | C8H8O3 |
![]() |
152.15 | 1000-5000 | 1.34 | N/A | N/A | ||
29617- 66-1 | α-chlorpropionic acid | ACPA/2- chloropropanoic acid | C3H5ClO2 |
![]() |
108.52 | freely soluble | 0.76 | 2.6 × 10-7 | N/A | ||
29617- 66-1 | i-chlorpropionic acid | LCPA/(S)-2- chloropropionic acid | C3H5ClO2 |
![]() |
108.52 | freely soluble | 0.76 | 2.6 × 10-7 | N/A | ||
50-21-5 | lactic acid | 2-hydroxypropanoic acid | C3H6O3 |
![]() |
90.08 | 100000 | -0.62 | 9.6 × 10-9 | N/A | ||
79-14-1 | glycolic acid | 2-Hydroxyethanoic acid | C2H4O3 |
![]() |
76.05 | 100000 | -1.11 | 1.09 × 10-4 | N/A | ||
104- 76-7 | 2-ethylhexanol | 2-ethylhexan-1-ol | C8H18O |
![]() |
130.23 | 1000 | 2.9 | 2.6 × 10-5 | N/A | ||
71-36-3 | n-butanol | butan-1-ol | C4H10O |
![]() |
74.12 | 68000 | 0.88 | 6.3 × 10-6 | N/A | ||
78-83-1 | i-butanol | isobutanol/2- methylpropan-1-ol | C4H10O |
![]() |
74.12 | 68000 | 0.88 | 6.3 × 10-6 | N/A | ||
108- 88-3 | toluene | Toluene | C7H8 |
![]() |
92.14 | 526 | 2.73 | 6.64 × 10-3 | N/A | ||
1330- 20-7 | total xylenes | dimethylbenzene | C8H10 |
![]() |
106.16 | 198 | 3.15 | 7.18 × 10-3 | N/A | ||
108- 21-4 | isopropyl acetate | propan-2-yl acetate | C5H10O2 |
![]() |
102.13 | 31900 | 1.28 | 2.78 × 10-4 | N/A |
Table 3: Chemical and physical properties of the chlorinated aromatic herbicides present in the pesticide production wastewater
Table 3 was generated using existing literature to present physical and chemical characteristics for the pollutants for the following constants; solubility, Log Kow, Kd, Hc, as well as the molecular weight and structure. These chlorinated herbicides (2,4-D, 2,4-DB, 2,4-DCP 2,4-DP, 2,4,6-TCP, PCOC, MCPA, MCPB and MCPP) have high molecular weights over 170 mg/L (except 2,4-DCP (163 mg/L) and PCOC (142.58 mg/L)), Log Kd values mostly above 1.5 and an octanol/water partition coefficient (Log Kow) over 2.5, indicating that these compounds have a hydrophobic nature and have a good adsorption capacity (Table 2). Therefore using a physical adsorption treatment such as granular activated carbon (GAC) could potentially remove the compounds in the wastewater. Chemical treatment processes such as advanced oxidation processes (AOPs) could potentially be used to treat the chlorinated herbicides as the compounds reactivity to hydroxyl radials (Log KoH) are around 109 1/M.s. However the fate of compounds with Log KoH values between 109 -1011 1/M.s are hard to interpret and experimental trials are frequently needed.
Biological Treatment
Biological processes are often used to treat industrial wastewater due to low operational and capital costs. However microorganisms are not always able to remove pesticides due to their recalcitrance, low water solubility or toxicity [4,27]. Organic pollutants can be degraded biologically either aerobically or anaerobically [28].
Aerobic treatment
Aerobic degradation of di-chlorinated pesticides, such as 2,4-D, is well understood, as these compounds have been released to the environment since the 1950’s [29]. Aerobic breakdown usually takes place by oxidation and cleavage of the esther bond and chlorophenol hydroxylation. The formed chloro-cathechol would then be modified by an ortho-cleavage pathway. Once the aromatic ring is open, the chlorinated acetate compound can be used in the normal microbial metabolism, the tricarboxylic acid cycle, and transformed into carbon dioxide and water.
Activated sludge processes
Although activated sludge processes are often used to treat low strength wastewater that contains biochemical oxygen demand (BOD) and chemical oxygen demand (COD)<1000 mg/L [30], these processes have been shown to be suitable to treat industrial wastewaters that contain COD from 500 mg/L to as high as 100,000 mg/L [31]. Generally this treatment process operates at 1-5 g/L mixed liquor suspended solids (MLSS) and has a sludge retention time (SRT) in the range of 8 to 25 days [32,4].
Many studies describe the difficulties of treating high strength wastewater containing toxic compounds such as; chlorinated phenols, chlorinated aromatic hydrocarbons (Table 4). Jin et al. [6] carried out a study treating pesticide wastewater using a pressurized activated sludge process with medium concentrations of COD (2500-5000 mg/L) (Table 4).
Process | Scale | Type of wastewater | Influent | Effluent | % Removal | Comments | Reference |
Activated sludge | Laboratory scale |
Landfill leachate |
2,4-D:1420 mg/L MCPA:2020 mg/L PCOC: 520 mg/L 2,4-DCP: 50 mg/L MCPB: 4 mg/L |
PCOC: 26 mg/l, MCPA & MCPB: 21 mg/L Chlorophenols (2,4-D, 2,4-DCP): 235 mg/L |
PCOC: 95% MCPA & MCPB: 99.5% Chlorophenol: 84% |
Reduced toxicity by 76%, Flow rate -177ml/h, HRT - 4.9 h, SRT- 34.9 h |
Mcallister et al. [12] |
Activated sludge with bioaugm- entation | Laboratory scale | Synthetic pesticide wastewater |
2,4-DCP: 24.7- 28.3 mg/L |
2,4-DCP: <15 mg/L |
60.2% (no bioaug.) 95.4% (bioaug.) |
HRT - 8 h; pH 7-7.8; 24h. Acclimatised sludge over a period of 6 months by synthetically adding 2,4-DCP |
Quan et al. [13] |
Pressurized activated sludge | Laboratory scale | Real pesticide wastewater (China) | COD: 2500- 5000 mg/L |
COD: 350-450 mg/L | 85%-92.5% COD in 6 h |
Aeration time 6 h Pressure 0.30 MPa Operation temp 25°C | Jin et al. [6] |
Activated sludge | Laboratory scale | Municipal wastewater |
MCPP: <0.36 µg/L |
<LOD - 0.18µg/L | 0% - 38% | pH 6.6-7.8 HRT 7-10 h |
Bernhard et al. [15] |
Trickling filters | Pilot scale | Dye production wastewater | COD: up to 36,000 mg/L | COD: 5000 mg/L | 60% - 70% | pH 5.5 -8.0. Operation cycle 24 h Aeration phase 23 h HRT 15 h Flow rate 500 m3/h |
Kornaros & Lyberatos [35] |
Trickling filters | Full scale | Real pesticide wastewater (full scale WWTP, UK) | Influent to trickling filters (in µg/L) 2,4-D: 220.39 2,4-DB: 13.67 2,4-DCP 2500.00 2,4-DP: 20.14 2,4,6-TCP: 87.40 MCPA: 155.18 MCPB: 17.89 MCPP: 64.24 |
(in µg/L) 2,4-D: 34.98 2,4-DB: 8.67 2,4-DCP 403.60 2,4-DP: 4.39 2,4,6-TCP: 20.47 MCPA: 29.14 MCPB: 10.11 MCPP: 11.33 |
2,4-D: 84% 2,4-DB: 37% 2,4-DCP: 84% 2,4-DP: 78% 2,4,6-TCP: 77% MCPA: 81% MCPB: 43% MCPP: 82% |
Average flow: 442 m3/day 28 units at 20 m width, 150 m length, 2 m depth Active filter bed area: 84000 m2& volume 168000 m3 Hydraulic loading rate: 0.42 m3/m2 BOD loading rate: 0.015 kg BOD/m3 |
Soares [36] |
Table 4: Overview of biological processes used to treat pesticide production wastewater
In this, the traditional activated sludge process was modified to create a pressurised aerated tank to overcome oxygen mass transfer. It was reported that when using a pressure at 0.30 MPa and an aeration time of 6h, the concentration of COD was reduced from 500-5000 mg/L to 230-370 mg/L, removing between 85-92.5% COD (Table 4). Mcallister et al. [12] and Quan et al. [13] used a laboratory scale continuous flow activated sludge unit, consisting of a partitioned aeration tank with a hydraulic retention time (HRT) of 4.9h and solid retention time (SRT) of 35h [12] and HRT of 8h [13]. In the study of Mcallister et al. [12] more than 84% of the recalcitrant pesticides (2,4-D, MCPA, PCOC, 2,4- DCP and 2,4,5-TCP) were removed from landfill leachate. Quan et al. [13] acclimatised the activated sludge in a conventional activated sludge system with and without bio augmentation over a period of 6 months to determine the removal percentage of 2,4-DCP. Results showed that the non bioaugumented system removed 60.2% 2,4-DCP whereas bio augmentation system removed up to 95.4% 2,4-DCP [13] (Table 4). McAllister et al. [12] did recommend a physical adsorption process such as an activated carbon (AC) as a post treatment process for the removal of residual total organic carbon (TOC) and toxicity from the activated sludge prior to discharge to the environment.
Trickling filters
Trickling filters are continuous fixed bed reactors that operate under aerobic conditions. Wastewater is trickled over the packing media allowing the wastewater to percolate through the media colonised by microorganisms. Over time, the bio film degrades the organic compounds in the wastewater.
Studies using trickling filters to degrade pesticides in wastewater are very limited [33,34]. Although literature shows trickling filters can be utilised to treat high strength wastewaters of 10,000 mg/L COD with removal efficiency between 60-70% [35], there is no information about pesticide treatment in this processes (Table 4). The only exception is the pesticide production wastewater treated at a UK full scale STW (Table 4). The area of active filter bed was 84000 m2 and the volume is 168,000 m3 . The hydraulic loading and BOD rates were 0.42 m3 /m2 .day and 0.015 kg BOD/m3 .day, respectively. The produced effluent had an average COD and BOD of 60.31 mg/L and 7.68 mg/L, respectively, presenting percentage removals of 64.8% for COD and 89.5% for BOD. The removals of pollutants were often above 75%, except for 2,4-DB with 36.6% removal and MCPB with 43.5%. Prior to discharge the effluent was further diluted with the STW effluent at an estimated 4-fold dilution [36].
Anaerobic treatment
Existing studies have reported the removal of chlorophenol pesticides including 2,4-DCP using anaerobic pathways [29]. Anaerobic breakdown usually takes place by reductive dehalogenation in which chlorine atoms are substituted by hydrogen atoms. Dehalogenation continues into lesserchlorinated phenols and further degraded into methane and carbon dioxide. More specifically, for 2,4-DCP the compound is mineralised via phenol, 4-hydroxybenzoic acid and benzoic acid [37].
Physical treatment
Physical treatments such as filtration, coagulation and adsorption and more specifically granular activated carbon (GAC) processes are exceptionally good at removing solids, specific pollutants with high octanol/water coefficient (Log Kow) and high adsorption-desorption coefficient (Kd ), usually indicating good adsorption. From the physical chemical properties of pesticide production wastewater charactisation discussed earlier (Table 3) the compounds 2,4-D, 2,4-DB, 2,4-DCP 2,4-DP, 2,4,6-TCP, PCOC, MCPA, MCPB and MCPP show potential to be removed via adsorption. Adsorption is often used for removal of recalcitrant pollutants due to its capacity, efficiency and applicability on a large scale and low costs [38-40]. The most effective and most frequently used adsorbent for organic removal in wastewater is activated carbon [41].
Activated Carbon
Activated carbon (AC) has been used extensively over the last 40 years to remove a wide range of persistent compounds from wastewater. With advantages such as its resistance to shock loads and efficient odour and colour removal and due to its highly developed surface properties such as porosity, surface area and surface chemistry [42-46]. Activated carbon is produced from carbonaceous sources such as coconuts, peat, coal and wood. The raw materials are activated by physical modification and thermal decomposition in a controlled temperature furnace. Once sufficiently burnt, the ash is activated using chemicals such as calcium chloride or zinc chloride. These chemicals create the pores inside each individual ash particle giving the final activated carbon product a large surface area per unit volume and a network of interlinking sub microscopic pores where adsorption will take place [47,48].
The physical adsorption process works by attracting the pollutants to stick onto the walls of the carbon. The adsorption mechanism happens in three stages. Firstly the pollutant adheres to the exterior surface of the activated particle due to the attractive forces. The pollutants continue to travel through the surface pores and travel deeper inside the AC, where the attractive forces are the strongest. This process continues to attract more pollutants until full capacity is reached. When this occurs the AC will need to be replaced with new AC or regenerated. Factors that affect the adsorption capacity are composition of the wastewater, pollutants being adsorbed, pH, temperature and contact time [49].
AC can be applied in a number of forms; the two most popular types are GAC and powder activated carbon (PAC). GAC particles have higher initial costs when compared to PAC, due to the size of the particles being large enough to be recovered and regenerated when the activated carbon has reached capacity. GAC is regenerated using thermal reactivation, which is an expensive process due to the high-energy costs. Even with GAC having higher initial costs and regeneration costs, from an economical perspective it has a greater adsorption capacity when comparing adsorption of 2,4-D against PAC and other AC media (Table 5). From literature, GAC is more likely to be used due the disadvantages of PAC mentioned [43,46]. PAC has a much lower initial cost as the particles are smaller in size and cannot be reused. Disadvantages of using PAC are clogging up and damaging expensive machinery if not retained in specific tanks or columns and the need to keep replacing and buying PAC as this cannot be re-used [48].
Table 5 shows that GAC, more specifically Filtrasorb 400 (a commercial GAC), is a more versatile product capable to removing range of different pesticides when compared to PAC. Maximum adsorption capacity of GAC for pesticides reached up to 516.8 mg/g whilst maximum adsorption capacity for PAC reached 333.3 mg/g, however this is dependent on the type pesticides been adsorbed. When comparing the adsorption capacity for 2,4-D, with GAC, PAC and date stones, the maximum adsorption capacity was 411.1 mg/g, 333.3 mg/g and 238.1 mg/g, respectively (Table 5). Filtrasorb 400 was able to remove more pesticides per gram of carbon used [47,50-52] (Table 5).
Process | Scale | Type of wastewater | Influent | Effluent | % Removal | Comments |
Reference |
Filtrasorb 400 (GAC) | Laboratory scale | Synthetic wastewater |
2,4-D: 0.45 mol/m3 MCPP: 0.45 mol/m3 Chlorophenoxyacetic acid: 0.45 mol/m3 |
2,4-D MCPP Chlorophenoxyacetic acid |
Batch tests: AC 0.001 – 0.25 g in 200 ml synthetic solution. Max adsorption capacity: 411.13, 389.20 and 516.85 mg/g Pesticide concentrations kept a constant 0.45 mol/m3 |
Kim et al. [47] |
|
AC – Tire granules | Laboratory scale | Synthetic wastewater | Methoxychlor: 12 mg/L Atrazine: 12 mg/L Methyl Parathion: 12 mg/L |
Methoxychlor: <1.2 mg/L Atrazine: <2.4 mg/L Methyl Parathion: <3.5 mg/L |
Methoxychlor: 91% Atrazine: 82% Methyl Parathion: 71% |
Column studies Particle size 200-250 µm Flow rate: 1.5 ml/min |
|
Filtrasorb 400 (GAC) | Laboratory scale | Synthetic wastewater |
Lindane: 10 mg/L Alachlor: 10 mg/L |
Batch tests: 3 days Particle Size: 0.84-1 mm Max adsorption capacity: Lindane: 181.00 mg/g, Alachlor: 151 mg/g |
Sotelo et al. [39] | ||
Charcoal based (PAC) | Laboratory scale | Synthetic wastewater | 2,4-D: 106-628 mg/L | 2,4-D: 97- 307 mg/L | 2,4-D: 91.9-48.8% | Batch tests, PAC 0.1 g in 100 ml 2,4-D solution at 25°C Max adsorption capacity: 333.30 |
Aksu & Kabasakal [53] |
AC - Date stones |
Laboratory scale | Synthetic wastewater | 2,4-D: 100 mg/L | 2,4-D: 30 mg/L | 2,4-D: 70% | Batch tests Particle Size: 2-3 mm AC 0.2 g in 250 ml solution Period of 9 hours Max adsorption capacity: 238.10 |
Hameed et al. [50] |
Table 5: Overview of pesticide absorption capacities for various types activated carbon
Aksu & Kabaskal [53] carried out batch studies comparing laboratory scale PAC treatment in 2,4-D in synthetic wastewater (Table 5). Aksu & Kabaskal [53] utilized PAC of 0.1 g in 100 ml solutions at 2,4-D concentrations of 106.0, 204.1, 416.8 and 628.6 mg/L and shaken for a period of 2 days at a temperature of 25°C. The results showed removal levels of 91.9%, 81.0%, 64.8% and 48.8%, respectively.
When pesticide concentrations are higher in the influent wastewater it often leads to lower removal efficiencies as the AC reaches its capacity in a shorter time. Also when treating real wastewater there will be competition for the GAC adsorption pores due to the real wastewater containing other contaminants such as other organic matter. This organic matter interferes with pesticide adsorption by been absorbed and reduces the GAC capacity, leading to lower removal yields [54].
Chemical treatment-advanced oxidation processes (AOPs)
Over recent decades, the number of studies on UV-based AOPs such as UV/H2O2 , O3 , UV/O3 , photocatalysis (UV/TiO2) or the Fenton process (H2O2 /Fe) is rapidly increasing due to their ability to remove persistent pollutants (Table 6) [20,8,10,11,23]. These new technologies have been acknowledged as extremely efficient at eliminating a wide range of organic compounds in wastewater including pesticides. Existing studies showed AOPs having the ability to degrade toxic pesticide compounds to less toxic compounds and even accomplished full mineralisation [55,22,56]. As a result more wastewater treatment plants are incorporating treatments using AOPs [57].
Processes | Scale | Type of wastewater | Influent | Effluent | % Removal | Comments |
Reference |
UV/TiO2 | Laboratory scale | Synthetic wastewater | PCOC: N/A | PCOC: N/A | 51.4% | pH 2.7 Reactor volume 130 ml 0.5 g/L TiO2 Max radiation 365 nm |
Irmak et al. [10] |
UV/H2O2 | Pilot scale | Real pesticide production wastewater (Poland) | 2,4-D: 65 µg/L | 2,4-D: <0.1 µg/L | 95% | 150 W medium pressure mercury vapour lamp. Irradiated for 40 minutes. UV/H2O2 Reactor volume 100L H2O2dose 0.8 v/v |
Kowalska et al. [11] |
Fenton | Laboratory scale | Synthetic wastewater | Fenitrothion: 50 mg/L Diazinion: 50 mg/L Profenofos: 50 mg/L |
Fenitrothion: 22.95 mg/L Diazinion: 43.55 mg/L Profenofos: 24.85 mg/L |
Fenitrothion: 54.1% Diazinion: 12.9% Profenofos: 50.3% |
Reactor volume 0.85L Optimal pH 3 Removal concentrations after 90 minutes |
Badawy et al. [20] |
Photo- Fenton |
Laboratory scale | Synthetic wastewater | Fenitrothion: 50 mg/L Diazinion: 50 mg/L Profenofos: 50 mg/L |
Fenitrothion: 6.55 mg/L Diazinion: 21.65 mg/L Profenofos: 5.15 mg/L |
Fenitrothion: 86.9% Diazinion: 56.7% Profenofos: 89.7% |
Reactor volume 0.85L UV Lamp 100-280 nm Optimal pH 3 Removal concentrations after 30 minutes |
Badawy et al. [20] |
UV/O3 | Laboratory scale | Synthetic wastewater |
Deltamethrin: 100 mg/L COD: 6500 mg/L |
Deltamethrin : <10 mg/L COD: 5200 mg/L |
Deltamethrin: 90- 100% COD: 20% |
pH 4 or above Ozonation 3.5 h 4200 mg ozone entered the oxidation reactor. UV emitted at 253 nm Ozone flow rate 240 mg/h/L |
Lafi & Al- Qodah [22] |
Ozonation | Laboratory scale | Synthetic wastewater | 2,4-D, 2,4-DP, MCPA, and MCPP 0.9- 6.4 µg/L | 2,4-D: >80% 2,4-DP: 86% MCPA: 100% MCPP: 100% |
Meijers et al. [56] |
||
Ozonation/ H2O2 | Laboratory scale | Synthetic wastewater |
2,4-D and 2,4-DP 0.9- 6.4 µg/L Atrazine: N/A |
2,4-D: >95% 2,4-DP:>96% Atrazine: 100% |
H2O2 /O3 >0.5g/g Temp 5-25°C Atrazine pH 7, 10 mins – 100% degradation Atrazine pH 8 , 1 min – 100% degradation |
Meijers et al. [56] |
|
Fenton | Laboratory scale | Mixture of pesticide production wastewater and landfill leachate (Poland) |
Organochlorine pesticides : Up to 377.1 µg/dm3 |
Organochlorine pesticides: N/A |
90 - 100% removed |
Completely degraded achieved only with H2O2concentration of 5 g/dm3. Optimum ratio of ferrous ion to H2O2 was between 1:2 and 1:3 with an optimum pH between 3- 3.5 |
Barbusiński & Filipek [8] |
Table 6: Overview of the AOP processes used for removal of pesticide from wastewater
Advanced oxidation processes use catalysts (titanium dioxide, iron ions, or other transition metals), oxidants (O3 or H2O2) and radiation (solar light, UV light or ultrasounds) either solely or combined. The most recognised and commercially available processes that are utilised for treating wastewater are ozonation (O3 ), ultra violet light with ozone (UV/O3) and ultra violet light with hydrogen peroxide (UV/H2O2) [58]. Photocatalysis describes accelerated photoreaction in the presence of a catalyst that is activated by light (the photocatalyst). The absorption of light causes the chemicals to change chemical states as molecules transfer electrons leading to the breakdown of pollutants into smaller compounds that are usually more easily degradable.
AOPs generate very powerful, non-selective hydroxyl radicals (HO• ) that are short lived [24,25]. These hydroxyl radicals degrade the organic compounds in the wastewater by oxidizing them. Organic compounds with second rate constants compound-radical between the order of 106 -109 1/M.s or higher, are likely to be oxidised by AOPs [22]. Rates of degradation are different for every compound and depend on the formation of oxidant species such as the radical scavengers in the wastewater as well as the hydroxyl radical. To overcome this and attain full mineralisation usually high amounts of chemicals are used. However this is impractical and exceptionally expensive especially when utilized on large scales [59].
Ozonation
Ozone is an unstable gas that readily degrades from O3 to O2 (Equation 1) to produce a highly reactive free radical that is stronger and less selective than chemical oxidants. Consequently ozone can successfully react and breakdown a wide range of organic pollutants and water (Equations 2 and 3). The mechanism for this process starts with the ozone generation. This is achieved by running an electric current through the air, which charges the molecules and converts O2 to O3. Ozone is introduced into contact tanks where it is dissolved in water. It can react directly with the pollutants or with water, yielding hydroxyl radicals. These free radicals are extremely unstable and short lived therefore they need to be generated in-situ. Due to the short reaction times ozonation allows a vast amount of wastewater to be treated in high throughput processes. Ozonation is a powerful oxidation technique that has long been used to treat water for odour management, disinfection and colour removal in the water treatment industry. Since then studies have been carried out and have proven ozonation is effective at degrading a number of recalcitrant organic pollutants including pesticides 2,4-D, 2,4-DP, MCPA, and MCPP in wastewater [56, 60,61].
3O2 + ENERGY → 2O3 (1)
2O3 + POLLUTANT → BY-PRODUCT (2)
O3 + H2 O → OH• ( 3)
Meijers et al. [56] utilised this process on the above compounds at concentrations between 0.9-6.4 µg/L, the removal rates were between 80- 100%. The study then continues looking at the effects of ozone combined with hydrogen peroxide for 2,4-D and 2,4-DP at the same concentrations between 0.9-6.4 µg/L the removal rates were >95% and >96%, respectively. These results indicate that when combining ozone with hydrogen peroxide significantly higher removal rates can be achieved (Table 6) [56].
Fenton process
The Fenton process is one of the most effective methods for oxidising organic pollutants in industrial wastewater. The Fenton reagent is constituted by a mixture of hydrogen peroxide (H2O2) and ferrous iron (Fe2+). The ferrous iron (Fe++) initiates and catalyses the decomposition of H2O2 , thus generating hydroxyl radicals (OH• ) (Equation 4). In this reaction ferrous iron is oxidised to ferric iron, which then reacts again with hydrogen peroxide, yielding more radicals (Equations 2-5). These two reactions constitute the iron redox cycle, where iron acts as a catalyst. The generation of hydroxyl radicals comprises of a complex sequence of reactions (Equation s 6 to 9). They can react with pollutants, oxidising and transforming them into by-products; they can react with other radicals; or with other ions/compounds in water (inefficient Equations). Hydrogen peroxide can also act as a hydroxyl scavenger as well as an initiator during these reactions (Equation s 7 to 9) [62].
Fe (photo) redox cycle: H2O2 + Fe2+ → Fe3+ + OH– + OH• (4)
Fenton: Fe3+ + H2O2 → OH• +HOO• + Fe2+ (5)
Fe2+ + OH• → Fe3+ + OH– (6)
OH• + H2O2 → H2 O + OOH• (7)
OOH• + Fe3+ → O2 + Fe2+ + H+ (8)
•OH + RH → R• + H2O → products (9)
The efficiency of Fenton’s oxidation process depends on hydrogen peroxide and ferrous ion concentrations, pH and time of the reaction. For Fenton process to work successfully, the pH should be between 2.5-4.0 [16]. In 2001, Barbusiński & Filipek [8] studied the impact of the Fenton’s process on the removal of organochlorine pesticides (concentrations upto 377.1 µg/L) in industrial wastewater. Using a hydrogen peroxide dose of 5 g/L at pH 3.0 - 3.5 all pesticides were completely removed [8] (Table 6).
In 2006, Badawy et al. [20] studied the effects of removing pesticides from a synthetic solution via Fenton and photo-Fenton treatment processes (Table 6). Using a reactor volume of 0.85 L and pH 3 and a 150 W medium pressure mercury lamp (100-280 nm). The results evidently indicate that the most effective method was the photo-Fenton process with higher removal rates between 57-90% in 30 minutes, compared to the Fenton process removal rates between 13-54% in 90 minutes [20].
Ultraviolet (UV) photolysis
UV light is a type of electromagnetic radiation with frequencies of around 8 × 1014 to 3 × 1016 cycles per second, known as hertz (Hz), and is categorised into four types: UV-A (near UV) between 315-400 nm; UV-B (middle UV) between 280-315 nm; UV-C (far UV) between 180- 280 nm and vacuum (extreme UV) between 10-180 nm. Different UV lamps can be used to treat wastewater: low pressure mercury lamps (LPUV -wavelength at 253.7 nm) are capable of providing power up to 0.4 kW and medium pressure lamps (MP-UV), can produce UV and visible light in the UV-C range of 180-280 nm and power up to 30 kW [63,20,64]. UV-C range is more commonly utilised when applied in combination with hydrogen peroxide [57].
When treating recalcitrant pollutants in wastewater, UV alone is not often used, as it is a slow process and efficient only for compounds absorbing light at the emitted wavelength of the lamp. So UV is commonly combined with hydrogen peroxide or ozone (Table 6) [11,22]. Combining either will generate more hydroxyl radicals that will bond and oxidise the pollutants in the wastewater and increase the rate of degradation. Other substances can be added such as catalysts such as titanium dioxide (TiO2), this also helps to increase the creation of hydroxyl radicals [10,16]. This treatment process is currently not been used at large scale, but studies have been carried out at bench/pilot scale work which would be easily scalable.
Ultraviolet/hydrogen peroxide (UV/H2O2)
Treatment processes based on hydrogen peroxide and UV (at 200-280 nm) generate high concentrations of hydroxyl radicals (OH) [57]. This process is the most studied AOP and the only one used at large scale. Studies show that UV/H2O2 process are extremely efficient in the removal of wide range recalcitrant compounds including organic pesticides and is currently used for pesticide removal in drinking water [11].
Kowalska et al. [11], studied the removal of organic pesticides including 2,4-D in industrial wastewater using UV/H2O2 (Table 6). In this study, filtration, sedimentation and coagulation were used as pre-treatment stages in order to reduce solids and enhance the pesticide removal efficiency at reduced irradiation, and therefore reduced costs. Kowalska et al. [11] used an air-sparged hydrocyclone reactor containing 100 L of industrial wastewater, doses of H2O2 of 80 mg/L, 40 minutes irradiation time and a 150 W MP-UV. The study showed the removal of 95% 2,4-D [11]. From earlier studies Kowalska et al. [11] established that the optimum dose of hydrogen peroxide was critical and must be experimentally determined [11]. Findings showed that at low hydrogen peroxide concentrations, the UV radiation instigates the generation of damaged hydroxyl radicals. Whereas at high concentrations of hydrogen peroxide, this acts as a trap to the hydroxyl radicals and compete with pollutants, reducing degradation efficiencies (Equation 10) [11].
HO· + H2O2 = HOO· + H2O (10)
Ultraviolet/ozone (UV/O3)
Treatment processes based on ultraviolet/ozone have a very high oxidation potential (2.8 eV). This high oxidation potential is greater than molecular ozone on its own, which means it can attack inorganic and organic molecules non selectively at very high reaction rates [60]. For this process to work efficiently certain parameters need to be carefully controlled such as, ozone dosage, UV irradiation level and pH. A high dissolved ozone rate must be sustained with an effective transfer of ozone gas into the wastewater. This can be achieved by using a pressurised injection mix UV/ozone reactor to produce micro bubbles that constantly top up the ozone gas. This type of reactor maintains and significantly improves the solubility of the gas allowing enhanced UV radiation. Furthermore, these set-up results in increased pH levels which force the ozone to produce additional hydroxyl radicals thus increase the oxidation rate [60]. In 2006, Lafi & Al-Qodah [22] used UV/O3 to remove pesticides and COD from aqueous solutions (Table 6). In this study, the aqueous solutions (100 mg/L Deltamethrin and 6500 mg/L COD) were adjusted to pH 4, UV was emitted at 253 nm and an ozonation period of 3.5 h with an ozone flow rate of 240 mg/Lh was used. The results showed pesticide removal between 90% and 100% over a period of 210 minutes. COD levels reduced by 20% but only at a pH of 4 or above [22].
Ozone/hydrogen peroxide (O3 /H2O2)
This combination of ozone/hydrogen peroxide is generally used in wastewater with very resistant pollutants or in very high concentrations that would result in the consumption of large amounts of oxidant. Ozone generation can be expensive and therefore combining with H2O2 makes the process more feasible [23]. During the reaction between ozone and H2O2 , hydroxyl radicals are formed (Equation 11). The stoichiometric ratio for the production of hydroxyl radicals between H2O2 and ozone is 0.35 g/g. For optimal formation of hydroxyl radicals the ratios are generally between 0.5-1 g/g [65].
2O3 + H2O2 → 2OH• + 3O2 (11)
Meijers et al. [56] investigated the degradation of pesticides by ozonation and advanced oxidation. They found that efficiency of hydroxyl radical formation via ozone and H2O2 process is determined by pH of the water and is relatively independent of the H2O2 dose (H2 O2 /O3>0.5 g/g) and temperature (5°C-25°C). Results showed that increasing the pH changes the oxidation mechanism from molecular ozone pathway (2.1 eV) to a radical oxidation potential (2.8 eV), therefore significantly increasing the rate of the reaction. For example, in the case of atrazine, at pH 7 the reaction took 10 minutes to degrade whereas at pH 8 the reaction was completed within 1 minute [56].
A full scale UV/ozone/peroxide treatment system for removing volatile organic compounds (VOCs) and chlorinated compounds was built and run for four years. The system was able to removed 94.6% VOCs. After 4 years the system was modified and improved to a UV/ H2O2 system. This system was able to achieve removals of 99.95% VOCs and 100% PCBs with initial influent concentration around 0.3 µg/L [66].
Hybrid treatment processes
Membrane bioreactor (MBR): Combining activated sludge with other biological/physical/chemical treatments processes enhances treatment efficiency. Activated sludge can be coupled with a membrane. A membrane bioreactor (MBR) is a hybrid treatment system that utilises an activated sludge process system with a membrane filtration in which the final settler is substituted by ultra-filtration or micro-filtration membranes, which retains all suspended solids therefore only allowing the clean effluent to pass through [4]. The membrane can be placed in various ways such as completely immersed or partially immersed in the activated sludge reactor. Submerged MBRs are currently replacing cross-flow MBRs due to being more energy efficient [67]. There are several membrane types available but for retaining particles between 1-5 nm nanofiltrations (NF) membranes are indicated, as these that can be used to retain dissolved particles. To retain particles sized between 5-100 nm ultrafiltrations (UF) membranes are indicated and these can also remove virus and smaller bacteria. For particle sizes between 100-1000 nm, i.e., suspended particles microfiltration (MF) membranes can be used. When treating high strength industrial wastewater shock loads might occur, for this reason MF are more commonly used allowing the retention of suspended solids, but not dissolved particles such as soluble microbial products, that would lead to membrane clogging and decrease the membrane life [68]. There are many advantages to using MBRs rather than conventional activated sludge systems including:
Small-foot print (usually 30-50%) due to higher biomass concentrations. Conventional ASP generally operates at 1-5 g/l MLSS, whilst the MBR operates significantly higher MLSS between 8-25 g/l, in some cases even higher concentrations allowing for higher reaction rates and consequently smaller foot-prints [4];
High effluent quality. MBR treatment eliminates all suspended solids and pathogens in the effluent, whilst standard activated sludge systems will require an additional tertiary processes to achieve the same effluent quality [67]
Simple process operation. MBRs are simple to operate, low sludge production. The long sludge retention times (SRT) in MBRs i.e., SRT for MBR are between 25-150 days compared to conventional treatment processes typically between 8-25 days [31,69,70,4] allows for sludge hydrolysis, reducing its production;
Enhanced pollutant removal. The sludge retained in the MBR can adsorb pollutants. It was found that the longer the SRT in the MBR, the more chance of microbial degradation of the pollutants [67]
MBR systems were originally used in treating domestic wastewater on various scales, [67] but over the last decades it has been developed and used for industrial wastewater treatment, including pesticides and pharmaceuticals. These wastewaters contain a wide spread of pollutants that are either slowly biodegradable or not biodegradable at all. Studies show that biodegradation rate is mostly affected by the adaptability of the microorganisms and the nutrients they get from the wastewater [71-73].
Yang et al. [74] and Bernhard et al. [15] carried out studies comparing laboratory scale MBR and activated sludge treatment in biodegradation of persistent organic pollutants (POPs) in pesticide wastewater (Table 7). Bernhard et al. [15] utilized a submerged MBR prototype with three chlorinated polyethylene membrane plates with a surface area of 0.1 m2 and pore size of 0.4 µm. The pH ranged between 6.6- 7.8 and the MBR had an HRT of 7-10h. The results showed that recalcitrant pollutants were completely removed. However MCPP removal percentage was between 36 - 64% [15,74].
Processes | Scale | Type of wastewater | Influent | Effluent | % Removal | Comments | Reference |
MBR | Laboratory scale | Domestic wastewater | MCPP: Unknown |
MCPP: <LOD - 0.10 µg/L |
38% - 64% | HRT 7-10 h Submerged MBR prototype with three membrane plates each with a surface area of 0.1 m2, mesh width of 0.4 µm. Membrane was chlorinated polyethylene | Bernhard et al. [14] |
MBR | Pilot scale | Real wastewater | Sulfamethoxazole: N/A | Sulfamethoxazole: N/A | 80% | HRT 13 h SRT 16 d, 30 d, 60-80 d Flow rate 1.3 m3/h |
Göbel et al. [69] |
Aerobic/ Anaerobic Biological Process | Laboratory scale | Synthetic wastewater | Triadimeno: 25 mg/L | Complete degradation | 96%+ | Aerobic: Acclimation 172 days HRT 24 h Operating temp 22°C Anerobic: Acclimation 230 days HRT 12 h Operating temp 30°C |
Shawaqfeh. [76] |
Activated Sludge electrochemical flow cell | Laboratory scale | Synthetic wastewater | 2,4-D: 30 mg/L (can be up to 500 mg/L) | 2,4-D: 10.2 mg/L |
66% in 2 days, 79% within 7 days, 85% within 21 days |
Porous electrode at a constant 1.6 V/SCE Flow rate of 1 mL/min. Stirred at 250 rpm at 30°. Inoculated with 0.5 g L-1 of activated sludge. pH 7 |
Fontmorin et al. [9] |
Photo-Fenton | Laboratory scale | Synthetic wastewater |
Fenitrothion: 50 mg/L Diazinion: 50 mg/L Profenofos: 50 mg/L |
Fenitrothion: 6.55 mg/L Diazinion: 21.65 mg/L Profenofos: 5.15 mg/L |
Fenitrothion: 86.9% Diazinion: 56.7% Profenofos: 50 mg/L 89.7% |
Reactor volume 0.85L UV Lamp 100-280 nm Optimal pH 3 Removal oncentration after 30 minutes |
Badawy et al. [20] |
Ozonation/ H2O2 |
Laboratory scale | Synthetic wastewater |
2,4-D & 2,4-DP: 0.9- 6.4 µg/L |
2,4-D & 2,4-DP: N/A |
2,4-D: > 95% 2,4-DP: >96% |
H2O2 /O3 >0.5 g/g Temp 5-25°C Atrazine pH 7 – 10 mins – 100% degradation Atrazine pH 8 – 1 min – 100% degradation |
Meijers et al. [56] |
Fenton/ Microwave electrodeless ultraviolet (MWEUV) | Laboratory scale | Real pesticide production wastewater (China) |
COD: 33,700 mg/L Dimethoate, Triazophos, and Malathion: N/A |
COD: 9300 mg/L Dimethoate, Triazophos, and Malathion: N/A |
COD: >85% Dimethoate, Triazophos, and Malathion: 100% |
Optimal conditions Fe2+ 0.8 mmol/L, H2O2 100 mmol/L, pH 5. 120 mins Temp 25°C |
Cheng et al. [55] |
IBR/Photo- Fenton/IBR |
Pilot scale | Domestic wastewater |
2,4-D: 33,552- 43,645 ug/l, MCPA:29,052- 38,752 ug/L |
2,4-D: <200 ug/L MCPA: <100 ug/L |
2,4-D: >99% MCPA: >99% |
Biological reactor volume 80 L Flow rate 250 L/h pH 6.5-7.5 Fenton Fe2+/ 140 mg/L every 15 mins pH adjusted to 2.6-2.9 200-500 mg/L H2O2 |
Vilar et al. [86] |
Immobilised biomass reactor (IBR)/UV/ Fenton, IBR)/ UV/TiO2 / H2 O2 and IBR/UV/TiO2 |
Pilot scale |
Wastewater resulting from phyto- pharma. Plastic containers washing |
2,4-D & MCPA : 25,000 ug/L |
2,4-D & MCPA : up to 2,500 ug/L |
2,4-D & MCPA : >90% |
Biological system flat bottom tank 50 L and IBR 45 L tank. pH 6.5-7.5 Air flow 20 L/min After 5-8 Kjuv/L. No pesticides were degraded after the IBR. IBR. Fenton – pH 2.6-2.9 140 mg Fe2+/L H2O2200-500 mg/L IBR-UV/TiO2 – up to 200 mg/L |
Moreira et al. [85] |
UV/O3/bioreactor | Laboratory scale | Synthetic wastewater | COD: 6500 mg/L | COD: <325 mg/L | COD: >95% | pH 4 or above. Ozonation was 3.5 hours. During this oxidation time, 4200mg ozone entered the oxidation reactor. Bioreactor 6 L tank Flow rate 200 L/h |
Lafi & Al- Qodah [22] |
TiO2 and Photo- Fenton/IBR |
Pilot scale | Synthetic wastewater |
Methomyl: 50 mg/L Dimethoate: 50 mg/L Oxamyl: 50 mg/L Cymoxanil: 50 mg/L Pyrimethanil: 50 mg/L |
Methomyl: 5 mg/L Dimethoate: 5 mg/L Oxamyl: 5 mg/L Cymoxanil: 5 mg/L Pyrimethanil: 5 mg/L |
Methomyl: >90% Dimethoate: >90% Oxamyl: >90% Cymoxanil: >90% Pyrimethanil: >90% |
TiO2 - 35 L solar pilot plant tank TiO2 - 200 mg/L Photo-Fenton-75 L solar pilot plant tank pH 2.7-2.9 Fe2+ 20 mg/L and 55 mg/L H2 O2 – 200-500 mg/L IBR - 60 L – neutralisation tank, 25 L conditioner tank, 35 L IBR tank pH 6.5-7.5 Flow rate 1.6 L/min |
Oller et al. [90] |
Photo-Fenton/ IBR |
Pilot scale | Synthetic wastewater | Pesticides: 500 mg/L | Pesticides: 0 mg/L | 100% removed | Photo-Fenton batch mode pH 2.7-2.9, 20 mg/L Fe2+. IBR - 1230 L tank pH 7-7.5. Batch or continuous Flow 120 L/h, HRT 20h |
Zapata et al. [87] |
Fenton/Activated Sludge |
Laboratory scale | Synthetic wastewater |
4- chlorophenol: 301- 313 mg/L |
4- chlorophenol:90-131 mg/L |
4- chlorophenol: <80% removed in 42 days |
Sample 200 ml Adjusted to pH 3 Fe2+ - 0.1- 14 g Temp 40°C, H2O2 – 17 mL (Fenton process 170 mins long) Adjusted to pH 6.5 Temp 25°C and activated sludge added. Samples kept in dark |
Kastanek & Maleterova, [88] |
Activated sludge, Moving Bed Bioreactor, Coagulation- Flocculation - UV, UV/ H2O2 , Fenton, Photo- Fenton |
Laboratory scale | Domestic wastewater |
MCPP: 235 ng/L (Activated sludge), MCPP:20 ng/L (Moving Bed Bioreactor), MCPP: 26 ng/L (Coagulation- Flocculation) |
MCPP: <58.75 ng/L (Activated sludge), MCPP:<5 ng/L (Moving Bed Bioreactor), MCPP: <6.5 ng/L |
100% removed by UV/ H O . 2 2 >25% removed by Fenton/photo- Fenton |
Micropollutants removed; Efficiency increased in following order: Coagulation- Flocculation (20%), Activated sludge (25%), Moving Bed Bioreactor (40%). AOPs UV/ H2O2 most efficient. UV-C – 254 nm (10-30 mins) H2O2 – 25 mg/L Fe2+ - 5 mg/L HRT – 4 h SRT – 2 d |
Giannakis et al. [64] |
GAC - TiO2 /UV | Pilot scale | Petro- chemical wastewater | 2,4 –DCP: 22 mg/L | 2,4 –DCP: 0 mg/L | 2,4 –DCP: 100% | 100% removal with 9 g/l Ti– GAC with 90 mins. Reactor tank – flow rate 32 L/h Contact time 30 mins Air flow rate 5 L/min Ti-GAC 112g |
Gu et al., [82] |
Activated Sludge electrochemical flow cell | Laboratory scale | Real wastewater | 2,4-D: 30-500 mg/L | 2,4-D: <330 mg/L | 2,4-D : 66% in 2 days, 79% within 7 days, 85% within 21 days |
Porous electrode at a constant 1.6 V/SCE Flow rate of 1 ml/min 100 mL of medium, stirred at 250 rpm, kept at 30°C Inoculated with 0.5 gL-1 of activated sludge. pH 7 |
Fontmorin et al. [9] |
Fenton/SBRs | Laboratory scale | Synthetic wastewater |
2,4-D and MCPA: 180 mg/L |
2,4-D and MCPA: 18 mg/L |
90% | Fenton pH 3 H2O2 /Fe2+ - 10:1 ratio SBRs - 3L SBR, Air flow 9 L/ min. Temp 30°C, 200 rpm and pH 7. Sequences of 8 h as follows: anoxic filling (1 h) aerated reaction (5.5 h) settling (1 h) and draw (0.5 h) HRT - 12 h |
Sanchis et al. [91] |
UV/TiO2 - biological |
Laboratory scale | Synthetic wastewater | 2,4-D: 800 mg/L | 2,4-D: 70-280 mg/L | 2,4-D : Photo-catalytic treatment - Up to 60% removed in 21 hrs. Bio treatment >90% removed between 20-24 hrs |
TiO2 - 1 g/L, UV – 256 nm pH 7 Biological- 50 mL sample 5% sludge – mixed overnight Temp – 30°C 200 RPM |
Samir et al. [92] |
Table 7: Overview of the combination of biological, physical and chemical processes (hybrid processes) used for removal of pesticide from wastewater
Sahar et al. [75] compared the differences in removal efficiencies between MBR and CAS for hydrophilic compounds trimethoprim and sulphamethoxazole, both have Kow valuesless than 1.0. Results showed that the MBR was more efficient due to hydrophilic compounds depending on biodegradation removal rather than been adsorbed on the biomass like hydrophobic compounds, providing the microorganisms can adapt with the compounds in the wastewater and by providing longer contact time MBR usually are able to biodegrade compounds better than conventional activated sludge [75].
Aerobic-anaerobic process: Literature shows limited research on using an anaerobic-aerobic process on pesticide wastewater at full scale. Shawaqfeh [76] studied the removal of pesticides from a synthetic wastewater using combined anaerobic-aerobic biological treatment. In this study, two small identical glass cylinders (height: 25 cm, diameter: 10 cm) were used for aerobic (22°C and HRT of 24h) and anaerobic conditions (30°C and HRT of 12h), respectively, and packed with 0.5 cm polyethylene terephthalate (PET) plastic beads with a diameter of 0.5 cm. The study showed the removal of 25 mg/L triadimenol (C14H18ClN3O2, chlorinated polycyclic aromatic hydrocarbon) by 96% after an acclimation period of 230 days in the anaerobic reactor and 172 days in the aerobic reactor [76]. The process increased biomass concentrations and the HRT was reduced from 12h to 8h (anaerobic) and 24h to 16h (aerobic) when compared to the individual processes. The results showed a removal of 98% in COD, showing that this hybrid process could treat pesticide wastewater [76].
GAC–biological process (pre-treatment or post-treatment): Oh and Tuovinen [77], studied the biodegradation of the phenoxy pesticides MCPP and 2,4-D in fixed film column reactors with GAC. Operating conditions were: 180 mL continuous flow reactor, a temperature at 22°C and an aeration rate of 200 ml/min. GAC was used as a biomass support matrix to grow the microbial biofilm. Results showed that 2,4-D was completely degraded in 4 days whilst MCPP efficiently removal was 88% at 7 days [77].
A full scale pesticide manufacturing company in Australia has developed a combined process that includes trickling filters, activated carbon and sequence batch reactors. There is limited data about the process or the results obtained. The trickling filters were used as a pre-treatment of an activated carbon system to remove the high COD levels before entering sequence batch reactors [78]. Other studies also show that biological process can be used prior to activated carbon to increase its efficiency and reduce costs. Using a biological process prior to activated carbon removes most of the competing organic matter and other easily biodegradable compounds in the wastewater allowing the activated carbon to adsorb the target pesticides and reduce frequency of regeneration [42,79, 40].
A more recent study showed a laboratory scale MBR combined with GAC (post treatment) in the removal of trace organic contaminants in synthetic wastewater. The MBR was seeded with AS from another MBR had been in continuous operation for over 3 years. The MBR operating conditions were: 24 hours HRT, a temperature of 20°C, a dissolved oxygen concentration of 3 mg/L and a pH in the range of 7.2-7.5. The GAC column operating conditions were: 7.5 g GAC and a flow rate of 2.4 ml/min (7 min EBCT). Results showed removal levels after the MBR were below 40% (fenoprop, naproxen, diclofenac, ketoprofen and carbamazepine), after GAC treatment removal efficiencies reached >98% [17]. Other studies show utilizing GAC as a post treatment is a viable option for eliminating trace organic from biological treated wastewater [54,80,81,18].
GAC-AOPs: More recent studies show the combination of GAC with chemical processes such as AOPs. Areerachakul et al. [49] combined GAC fixed bed adsorption with a continuous photocatalysis system to remove pesticides from wastewater. In this study, combinations of columns were used with different bed depths (5, 10, 15 cm) packed with GAC and operated at empty bed contact times for several weeks. The removal of metsulfuron-methyl reached 35, 55 and 65% respectively. When using a continuous photocatalysis system using TiO2 the results showed removal rates between 40-60%. However when combining both processes GAC followed by photocatalysis system using TiO2 , the results showed removal rates of over 90% with a retention time of less than 10 minutes [49]. TiO2 is a technology under development and there is limited research on the process been scaled up. Gu et al. [82], confirms the success of hybrid systems where utilizing GAC with supported TiO2 and photocatalysis to degrade 2, 4-D is very efficient with 100% removal within 90 minutes [82] (Table 7).
Other studies report the utilisation of ozonation as a pre-treatment prior to GAC, this is so all remaining organic compounds and byproducts of degradation can be completely removed before discharged [83]. Other studies have combined activated sludge with ozonation treating real wastewater at pilot scale (Table 7). Existing literature supports pre-treatment rather than post treatment due to the positive impact on removing the remaining pollutants in the following biological treatment, such as activated sludge. However if treating strongly polluted wastewater, high dosing would be required, and consequently usually ozonation is deemed economically unfavourable due to the high cost implications. Therefore if using ozonation on a large scale with highly polluted wastewater post ozonation would be the preferable option or other treatment options would be used [82, 22].
Biological-AOPs: Existing literature indicates promising results using AOPs as a pre-treatment for degrading pesticides into more readily biodegradable intermediates which can then be fully treated by a biological treatment process [21, 84].
Research shows an increase in studies for combining Fenton process with other processes such as biological treatments, UV and photo-catalysis, to reduce costs, enhance pesticide removal and reduce toxicity (Table 7) [7,64,85-87]. Treating pesticide wastewater using a combined biological and solar driven Fenton process is a highly efficient process. Using UV lamps are also efficient but would give very different results because of the emitting spectrum. Moreira et al. [85] combined an immobilised biomass reactor (IBR) as pre-treatment biological process step using a flat bottom tank (50 L) and IBR tank (45 L) at pH 6.5-7.5 and an air flow rate of 20 L/ min with several different AOPs including Fenton, UV, TiO2 /UV (with and without acidification) and the processes were repeated with the addition of hydrogen peroxide. The Fenton process was controlled at pH 2.6-2.9, 140 mg Fe2+/L and H2O2 200-500 mg/L. TiO2 concentrations were added up to 200 mg/L. They found that TiO2 /H2O2 /UV with acidification was the most successful, not only did the process remove the highest pesticide removal efficiency of over 90% including recalcitrant pesticides 2,4-D and MCPA the rate of reaction was much quicker; 18 out of the 19 pesticides were degraded within 28K Juv/L. However their research indicated that no pesticides were degraded after the IBR (Table 7) [85].
Kastanek & Maleterova [88] used Fenton process as a pre-treatment combined with an aerobic activated sludge biological treatment to completely degrade 4- chlorophenol in wastewater (Table 7). 4-chlorophenol is from the same chemical group as 2,4-DCP and have very similar characteristics and this was chosen as model compound due to the limited biodegradability. The aim of this study was to utilise the Fenton process to modify the chemical structure of 4-chlorophenol with the purpose of generating biodegradable intermediates which would enhance the biodegradability. The Fenton process conditions were 200 mL samples at pH 3 at a temperature of 40°C, with doses of Fe2+-0.1- 14 g and H2O2 -17 mL and stirred for 170 minutes. After the pH was adjusted to 6.5 and activated sludge was added. The samples were kept at 25°C in the dark overnight. The results showed up to 80% 4-chlorophenol removal. By using a combination of Fenton reagent and a biological treatment process to remove recalcitrant pesticides offers economical and feasible advantages [88]. Combining with a biological process reduces costs and the amount of chemicals required [22,84].
Biological- Electrochemical
Fontmorin et al. [9] combined an electrochemical system with a biological treatment to study the removal 2,4-D in real pesticide wastewater at laboratory scale. In this study an electrochemical flow cell was used for pre-treatment using a graphite felt as an electrode (48 mm diameter and 12 mm width) and was kept at a constant 1.6 V/SCE and a flow rate of 1 ml/min. Biological experiments were carried out in 250 mL conical flasks containing 100 mL activated sludge and stirred at 250 rpm at 30°C. The study showed the removal of 30 mg/L 2,4-D by 66% in 2 days, 79% within 7 days and 85% within 21 days [9].
AOPs - Ultrasonic
Ozonation has been studied in combination with chemicals oxidants such as potassium permanganate, chlorine dioxide, and hydrogen peroxide. However molecular ozone has a higher oxidation potential of 2.07 V than conventional chemical oxidants listed above [60]. In 2011, Xiong et al. [89] studied pre-treatment of heterocyclic pesticide wastewater combining ultrasound and ozone processes. In this study, 100 mL pesticide wastewater was treated with ultrasound (power 300 W at 20-60 kHz) and dosage of O3 of 454.8 mg/L.min. The results showed significantly enhanced biodegradability and decreased levels of toxicity (from 11% to 52%). BOD5 /COD ratio increased from 0.03 to 0.55. It was found that the process was more favourable in alkaline conditions (pH 9) and the COD removal was improved when the ultrasound frequency was low (20 kHz) [89].
AOPs-AOPs
Studies show that coupling UV/H2O2 with the photo Fenton process is also a remarkably efficient treatment process. Badawy et al. [20] used this process and compared it with the Fenton process for the removal of organo-phosphorus pesticides in wastewater. The aim was to remove 3 different compounds, fenitrothion, diazinion and profenofos. Using a reactor volume of 0.85 L and pH 3, for the photo-Fenton process a 150 W medium pressure mercury lamp was used between 100-280 nm. The results showed that Fenton treatment removed 54.1%, 12.9% and 50.3%, respectively after 90 minutes, whereas combining UV/H2O2 with the Fenton treatment the results were 86.9%, 56.7% and 89.7%, respectively after 30 minutes. UV/H2O2 with the Fenton treatment is found to be much more efficient giving higher percentage removal and at a much quicker rate. The reason for these results can be explained looking at Equations 2-12. Equation 4 shows the Fenton process reaction, one ferrous ion (Fe2+) generates one hydroxyl radical (OH•) therefore ferrous ion can potentially be a limiting factor and affect the rate of hydroxyl radicals been produced therefore decrease the rate of degradation. Whereas in the UV/H2O2with the Fenton treatment process (Equation 12) the UV radiation contributes by photolysis of Fe3+ complex ions and the hydrogen peroxide to generate hydroxyl radicals. The hydrogen peroxide also reduces Fe2+ from the photolysis of Fe3+ which in turn is oxidised by hydrogen peroxide and produces further hydroxyl radicals therefore speeds up the oxidisation of the organic compounds [20].
Fe3+ + H2O2 + hν → Fe2+ + OH• + H+ (12)
Pesticide production wastewater is a high strength complex wastewater that requires bespoke treatment before it can meet industrial consent to discharge to sewer or meets consent to discharge direct to watercourse (rivers/streams). The Environmental Quality Standards (EQS) set the discharge limits for this specific wastewater, which are; 7.1 mg/L (2,4, dichlorphenoxy acetic acid: 2,4-D), 73 mg/L (2,4 dichlorophenol: 2,4- DCP), 219 mg/L (mecoprop: MCPP) and 70.2 mg/L (4-chloro-2-methyl phenoxyacetic acid: MCPA).
2,4, dichlorphenoxy acetic acid (2,4-D); 4-(2,4 dichlorphenox) propionic acid (2,4-DP); 4-(2,4, dichlorophenox) butyric acid (2,4- DB); 2,4 dichlorophenol (2,4-DCP); 2,4,6-trichlorophenol (2,4,6- TCP); 4-chlororthocresol (PCOC); 4-chloro-2-methyl phenoxyacetic acid(MCPA), 4-(4-chloro-2-methylphenoxy) butyric acid (MCPB) and mecoprop (MCPP)
A large proportion of the pollutants in pesticide production wastewater such as lactic acid, glycolic acid, 2-ethylhexanol, n-butanol, i-butanol, isopropyl acetate are easily biodegradable and can easily treated using biological processes. These pollutants are likely to be responsible for providing the highest pollutant load in the pesticide production wastewater measured as BOD. Hence it is typically recommended that a biological process is included on the possible options to treat the wastewater. After extensive review of the existing literature regarding treatment of pesticide rich wastewater (Tables 3 to 7) it is clear that activated sludge can successfully achieve pesticide removal between 0-99.5% for the following compounds 2,4, dichlorphenoxy acetic acid (2,4-D); 4-(2,4 dichlorphenox) propionic acid (2,4-DP); 4-(2,4, dichlorophenox) butyric acid (2,4-DB); 2,4 dichlorophenol (2,4-DCP); 2,4,6-trichlorophenol (2,4,6-TCP); 4-chlororthocresol (PCOC); 4-chloro-2-methyl phenoxyacetic acid(MCPA), 4-(4-chloro2-methylphenoxy) butyric acid(MCPB) and mecoprop (MCPP)in concentrations from 0.00036 - 2020 mg/L at laboratory scale [15,12]. At a full scale wastewater treatment plant (WWTP)where trickling filters have been used to treat pesticides 2,4-D, 2,4-DB 2,4-DP, 2,4-DCP, 2,4,6-TCP, PCOC, MCPA, MCPB and MCPP, pesticide removals have been observed in the range of 37-84% with initial concentration in the range of 14- 2500 µg/L [36]. Suggesting that pesticide production wastewater should be able to be treated by biological processes such as activated sludge, or more effectively even, by using membrane bioreactor (MBR) systems. Membrane bioreactors have the potential to improve the treatment of pesticide wastewater as it can reach higher effluent qualities and most importantly it has increased of resilience to toxic loads as a results more studies are been reported. These MBR systems were originally used in treating domestic wastewater at various scales but over the last decades MBRs have been developed and used for industrial wastewater treatment including pesticides and pharmaceuticals [67].
From existing literature it has been shown that 2,4-D, 2,4-DP, MCPA, PCOC and MCPP can be removed by various advanced oxidation processes (AOPs) in synthetic and real pesticide wastewater at laboratory and pilot scale with pesticide removals in the range of 50-100% [20,26,8,65,11,22,58]. However there are no reports on fullscale applications and there are very limited reports on commercial processes. On the other side physical treatments such as activated carbon (AC) appear to be a treatment option that has been applied by different industries. Looking at the field of pesticides treatment, it has been proven that granular activated carbon (GAC), more specifically F-400, can remove recalcitrant pollutants including 2,4-D and MCPP at levels of 100% and 88%, respectively. However when looking to treat pesticide wastewater that contains high concentrations (mg/L) it has been seen that higher concentrations in the influent wastewater often leads to lower removal efficiencies as the AC reaches its capacity in a shorter time. Also when treating real wastewater there will be competition for the GAC adsorption pores due to the real wastewater containing other contaminants such as other organic matter. This organic matter interferes with pesticide adsorption by being absorbed and reduces the GAC capacity, leading to lower removal yields [54].
On the other hand, even if the average pesticide production removals of 99% are achieved in the biological, chemical and physical processes, this would still result in effluent qualities with total pesticide concentration (sum of all 2,4-D; 2,4-DP; 2,4-DB; 2,4-DCP; 2,4,6-TCP; PCOC; MCPA; MCPB AND MCPP) in the order between 1-5 mg/L and compliance with the WFD EQS would be a risk of been breached. The majority of existing literature focuses on pesticide wastewater at low pesticide concentrations in the range of µg/L and usually in mixtures of one to four different pesticides; the more common pesticides 2,4-D, 2,4-DCP, MCPA and PCOC [9-13].
Therefore an application of a hybrid system utilising biological treatment with pre/post treatment chemical or physical would be necessary to ensure that these pesticides are removed to the required limits prior to being discharged. Research suggests that using a hybrid processes combining biological processes with physical and chemical processes are extremely successful in treating pesticide wastewater and some studies have proven to achieve complete mineralisation. Advanced oxidation processes (AOPs) can be combined with a biological process as a pre or post treatment stage. Many studies utilise activated sludge process with UV/H2O2, Fenton, photo-Fenton, O3 , UV/O3, UV/O3 /H2O2 . Studies show that utilising a hybrid process increases the removal of pesticides [20, 7,55,24,64,82,60,93-95,23,85,16,57]. On the down side, most of the processes described to date on the combination of AOPs with a biological process have been proven at lab-scale or pilot-scale. Applications at full-scale are very scarce and important parameters, such as process design, operation and costs would require further trials at a demonstration scale.
The vast majority of studies focus on low pesticide concentrations in synthetic and real wastewater, but the pesticide production wastewater has a very high strength wastewater (mg/L) that contained many toxic pollutants. The characterisation of the pesticides physical and chemical properties, existing literature and current commercial treatments show that these chlorinated herbicides (2,4-D, 2,4-DB, 2,4-DCP 2,4-DP, 2,4,6- TCP, PCOC, MCPA, MCPB and MCPP) can be removed by biological processes but potentially not to the EQS discharge limits. The pesticides have a hydrophobic nature and good adsorption capacities (sludge distribution coefficient (Kd) above 1.5 and octanol/water coefficient (Log Kow) over 2.5) therefore using a physical adsorption treatment such as GAC would further remove the pollutants in the wastewater (up to 99.9%) and provide a cheaper and more reliable alternative treatment option to AOPs, [96] as the fate of the pollutants is unclear due to the reactivity to hydroxyl radials (Log KoH) values been around 109 1/M.s. Also there is very limited data on full scale processes. Existing literature shows GAC has been commercially applied by different industries (GHD, 2015) and studies show that using GAC as a post-treatment as opposed to pre-treatment has more advantages such as; regeneration becomes less frequent, removal rates increase and reaction time is the order of minutes. Further to this, GAC has the ability to resist shock loads and can improve odour and colour removal [41-43]. Therefore combining GAC as a posttreatment with a biological process such as an MBR would have more advantages by reducing operation and set up costs and produce a high quality effluent that would comply with the EQS discharge limits.
Most studies found in literature focused on the fate of pesticides in synthetic solutions, synthetic wastewater, at lab-scale or pilotscale. Although these studies can provide information on the removal mechanisms and provide a comparison between process efficiency, they have limited practical applicability. The process that has been more widely used to treat high strength wastewaters rich in recalcitrant compounds at full-scale, is the combination of biological/GAC and GAC/biological processes. The pesticide production wastewater contains a variety of compounds, that can be removed by 80-90% using biological processes (such as MBR) and GAC has been shown to selectively remove the pesticides, potentially creating a high quality effluent. Nevertheless, in order to assert processes design, efficiencies or costs, it is crucial to evaluate these processes experimentally.
- Sahu O (2014) Treatment of Pesticides Industry Wastewater by Water Hyacinth (Eichornia crassipes) Adv Res J Biochem Biotechnology 1: 6-13. [Ref.]
- Misra R, Satyanarayan S, Potle N (2013) Treatment of agrochemical / Pesticide Wastewater by Coagulation / Flocculation Process. Int J Chem Phy Sci 2: 39-51. [Ref.]
- Defra (2014) Water Framework Directive implementation in England and Wales: new and updated standards to protect the water environment: 41. [Ref.]
- Cirja M, Ivashechkin P, Schufer A, Corvini PFX (2008) Factors affecting the removal of organic micropollutants from wastewater in conventional treatment plants (CTP) and membrane bioreactors (MBR). Rev Environ Sci Biotechnol 7: 61-78. [Ref.]
- IFC (1998) Pesticides Manufacturing. 367-370. [Ref.]
- Jin Z, Pan Z, Yu S, Lin C (2010) Experimental study on pressurized activated sludge process for high concentration pesticide wastewater. J Environ Sci 22: 1342-1347. [Ref.]
- Cheng H, Xu W, Liu J, Wang H, He Y, et al. (2007) Pretreatment of wastewater from triazine manufacturing by coagulation, electrolysis, and internal microelectrolysis. J Haz Mat 146: 385-392. [Ref.]
- Barbusiński K, Filipek K (2001) Use of Fenton’s Reagent for Removal of Pesticides from Industrial Wastewater. Pol J Environ Stud 10: 207- 212. [Ref.]
- Fontmorin JM, Fourcade F, Geneste F, Floner D, Huguet S, et al. (2013) Combined process for 2,4-Dichlorophenoxyacetic acid treatment-Coupling of an electrochemical system with a biological treatment. J Biochem Eng 70: 17-22. [Ref.]
- Irmak S, Kusvuran E, Erbatur O (2004) Degradation of 4-chloro-2- methylphenol in aqueous solution by UV irradiation in the presence of titanium dioxide. Appl Catal B: Environ 54: 85-91. [Ref.]
- Kowalska E, Janczarek M, Hupka J, Grynkiewicz M (2004) H2O2/UV enhanced degradation of pesticides in wastewater. Water Sci Technol 49: 261-266. [Ref.]
- Mcallister PJ, Bhamidimarri SMR, Chong R, Manderson GJ (1993) Activated sludge treatment of phenoxy herbicides and chlorophenols. Water Sci Technol 28: 111-115. [Ref.]
- Quan X, Shi H, Liu H, Lv P, Qian Y (2004) Enhancement of 2,4-dichlorophenol degradation in conventional activated sludge systems bioaugmented with mixed special culture. Water Res 38: 245-253. [Ref.]
- Moreira FC, Vilar VJP, Ferreira ACC, dos Santos FRA, Dezotti M, et al. (2012) Treatment of a pesticide-containing wastewater using combined biological and solar-driven AOPs at pilot scale. J Chem Eng 209: 429-441. [Ref.]
- Bernhard M, Muller J, Knepper TP (2006) Biodegradation of persistent polar pollutants in wastewater: Comparison of an optimised labscale membrane bioreactor and activated sludge treatment. Water Research 40: 3419-3428. [Ref.]
- Oller I, Malato S, Sanchez-Perez JA (2011) Combination of Advanced Oxidation Processes and biological treatments for wastewater decontamination-A review. Sci Total Environ 409: 4141-4166. [Ref.]
- Evoqua Water Technologies (2016) Organic Chemicals Removal from Groundwater. [Ref.]
- Nguyen LN, Hai FI, Kang J, Price WE, Nghiem LD (2012) Removal of trace organic contaminants by a membrane bioreactor-granular activated carbon (MBR-GAC) system. BioresourTechnol 113: 169-173. [Ref.]
- Melin T, Jefferson B, Bixio D, Thoeye C, De Wilde W, et al. (2006) Membrane bioreactor technology for wastewater treatment and reuse. Desalination 187: 271–282. [Ref.]
- Badawy MI, Ghaly MY, Gad-Allah TA (2006) Advanced oxidation processes for the removal of organophosphorus pesticides from wastewater. Desalination 194: 166-175. [Ref.]
- Irmak S, Yavuz, HI, Erbatur O (2006) Degradation of 4-chloro-2- methylphenol in aqueous solution by electro-Fenton and photoelectroFenton processes. Applied Catalysis B: Environmental 63: 243-248. [Ref.]
- Lafi WK, Al-Qodah Z (2006) Combined advanced oxidation and biological treatment processes for the removal of pesticides from aqueous solutions. J Hazar Mater 137: 489-497. [Ref.]
- Mokrini A, Ousse D, Esplugas S (1997) Oxidation of Aromatic Compounds with UV Radiation/Ozone/Hydrogen Peroxide. Water Sci Technol 35: 95-102. [Ref.]
- Comninellis C, Kapalka A, Malato S, Parsons SA, Poulios I, et al. (2008) Advanced oxidation processes for water treatment: Advances and trends for R&D. J Chem Tech Biotechnol 83: 769-776. [Ref.]
- García-Montaño J, Ruiz N, Muñoz I, DomènechX, García-Hortal JA, et al. (2006) Environmental assessment of different photo-Fenton approaches for commercial reactive dye removal. J Hazar Mater 138: 218-25. [Ref.]
- Barbusiński K (2005) Toxicity of Industrial Wastewater Treated by Fenton’s Reagent. Pol J Environ Sci 14: 11-16. [Ref.]
- Lapertot M, Pulgarin C, Fernandez-Ibanez P, Maldonado MI, PerezEstrada L, et al. (2006) Enhancing biodegradability of priority substances (pesticides) by solar photo-Fenton. Water Research, 40: 1086-1094. [Ref.]
- Zheng C, Zhao L, Zhou X, Fu Z, Li A, et al. (2013) Treatment Technologies for Organic Wastewater. In Tech 38. [Ref.]
- Maltseva O, McGowan C, Fulthorpe R, Oriel P (1996) Degradation of 2,4-dichlorophenoxyacetic acid by haloalkaliphilic bacteria. Microbiol 142: 1115–1122. [Ref.]
- Grady LCP, Daigger GT, Love NG (2011) Biological wastewater treatment. Third Edition CRC Press. [Ref.]
- Orhon D, Babuna FG, Karahan O (2009) Industrial wastewater treatment by activated sludge. IWA Publishing. [Ref.]
- Stephenson T, Brindle K, Judd S, Jefferson B (2000) Membrane bioreactors for wastewater treatment. IWA Publishing. [Ref.]
- Butler CS, Boltz JP (2014) 3.6 – Biofilm Processes and Control in Water and Wastewater Treatment. Comprehensive Water Quality and Purification 3: 90–107. [Ref.]
- Simsek H, Kasi M, Ohm JB, Blonigen M, Khan E, et al. (2013) Bioavailable and biodegradable dissolved organic nitrogen in activated sludge and trickling filter wastewater treatment plants. Water Res 47: 3201-10. [Ref.]
- Kornaros M, Lyberatos G (2006) Biological treatment of wastewaters from a dye manufacturing company using a trickling filter. J Hazar Mater 136: 95-102. [Ref.]
- Soares A (2015) Pesticide production wastewater treatment: Assessment of current treatment and other possible treatment options.
- Arora PK, Bae H (2014) Bacterial degradation of chlorophenols and their derivatives. Microbial Cell Factoriesn 13: 31. [Ref.]
- Daneshvar Nezamaddin, ASKARMH (2007) Investigation of adsorption kinetics and isotherms of imidacloprid as a pollutant from\r\ naqueous solution by adsorption onto industrial granular activated carbon. J FoodAgriculEnviron5: 425–429.
- Sotelo JL, Ovejero G, Delgado JA, Martınez I (2002) Adsorption of ́ lindane from water onto GAC: effect of carbon loading on kinetic behavior. Chem J Engineering 87: 111-120. [Ref.]
- Salman JM, Hameed BH (2010) Adsorption of 2,4-Dichlorophenoxyacetic Acid and Carbofuran Pesticides onto Granular Activated Carbon. Desalination 256: 129-135. [Ref.]
- Aksu Z, Kabasakal E (2004) Batch adsorption of 2,4-dichlorophenoxyacetic acid (2,4-D) from aqueous solution by granular activated carbon. Separation and Purification Technology 35: 223-240. [Ref.]
- Bonvin F, Jost L, Randin L, Bonvin E, Kohn T (2016) Superfine powdered activated carbon (SPAC) for efficient removal of micropollutants from wastewater treatment plant effluent. Water Research 90: 90-99. [Ref.]
- Foo KY, Hameed BH (2010) Detoxification of pesticide waste via activated carbon adsorption process. J Hazar Mater 175: 1-11. [Ref.]
- Knopp G, Prasse C, Ternes T, Cornel P (2016) Elimination of micropollutants and transformation products from a wastewater treatment plant effluent through pilot scale ozonation followed by various activated carbon and biological filters. Water Res100: 580- 592. [Ref.]
- Mailler R, Gasperi J, Coquet Y, Derome C, Buleté A, et al (2016) Removal of emerging micropollutants from wastewater by activated carbon adsorption: Experimental study of different activated carbons and factors influencing the adsorption of micropollutants in wastewater. J Environ Chem Eng 4: 1102-1109. [Ref.]
- Martinez ML, Torres MM, Guzman CA, Maestri DM (2006) Preparation and characteristics of activated carbon from olive stones and walnut shells. Industrial Crops and Products 23: 23-28. [Ref.]
- Kim TY, Park SS, Kim SJ, Cho SY (2008) Separation characteristics of some phenoxy herbicides from aqueous solution. Adsorption 14: 611. [Ref.]
- Chemviron Carbon (2016) Powdered activated carbon. [Ref.]
- Areerachakul N, Vigneswaran S, Ngo HH, Kandasamy J (2007) Granular activated carbon (GAC) adsorption-photocatalysis hybrid system in the removal of herbicide from water. Separation and Purification Technology 55: 206-211. [Ref.]
- Hameed BH, Salman JM, Ahmad AL (2009) Adsorption isotherm and kinetic modeling of 2, 4-D pesticide on activated carbon derived from date stones. J Hazar Mater 163: 121-126. [Ref.]
- Sotelo JL, Ovejero G, Delgado JA, Martínez I (2002) Comparison of adsorption equilibrium and kinetics of four chlorinated organics from water onto GAC. Water Res 36: 599-608. [Ref.]
- Sotelo JL, Ovejero G, Delgado JA, Martínez I (2002) Comparison of adsorption equilibrium and kinetics of four chlorinated organics from water onto GAC. Water Res 36: 599-608. [Ref.]
- Aksu Z, Kabasakal E (2005) Adsorption Characteristics of 2,4-Dichlorophenoxyacetic Acid (2,4-D) from Aqueous Solution on Powdered Activated Carbon. J Environ Sci Health B 40: 545-570. [Ref.]
- Alrhmoun M, Casellas M, Baudu M, Dagot C (2014) Efficiency of Modified Granular Activated Carbon Coupled with Membrane Bioreactor for Trace Organic Contaminants Removal. Int J Chem Mol Nucl Mater and Metal Eng 8: 43-49. [Ref.]
- Cheng G, Lin J, Lu J, Zhao X, Cai Z, et al. (2015) Advanced Treatment of Pesticide-Containing Wastewater Using Fenton Reagent Enhanced by Microwave Electrodeless Ultraviolet. BioMed Res Int [Ref.]
- Meijers RT, Oderwald-Muller E, Nuhn PANM, Kruithof JC (1995) Degradation of pesticides by ozonation and advanced oxidation. Ozone: Sci Eng 17: 673-686. [Ref.]
- Cesaro A, Naddeo V, & Belgiorn V (2013) Wastewater treatment by combination of advanced oxidation processes and conventional biological systems. J Bioremed Biodegrad 4: 222–230. [Ref.]
- Suty H, De Traversay C, Cost M (2004). Applications of advanced oxidation processes: present and future. Water Sci Technol 49: 227– 233. [Ref.]
- Wu C, Linden KG (2008) Degradation and byproduct formation of parathion in aqueous solutions by UV and UV/H202. Water Res 42: 4780-4790. [Ref.]
- Ikehata K, Gamal El-Din M (2005) Aqueous Pesticide Degradation by Ozonation and Ozone-Based Advanced Oxidation Processes: A Review (Part II). Ozone: Sci & Eng 27: 173-202. [Ref.]
- Alvares ABC, Diaper C, Parsons SA (2001) Partial Oxidation by Ozone to Remove Recalcitrance from Wastewaters - a Review. Environ Tech 22: 409-427. [Ref.]
- Pignatello JJ, Oliveros E, MacKay A (2006) Advanced Oxidation Processes for Organic Contaminant Destruction Based on the Fenton Reaction and Related Chemistry. Critical Reviews in Environ Sci Technol 36: 1-84. [Ref.]
- Zhang Y, Pagilla K (2010) Treatment of malathion pesticide wastewater with nanofiltration and photo-Fenton oxidation. Desalination 263: 36- 44. [Ref.]
- Giannakis S, Gamarra Vives FA, Grandjean D, Magnet A, De Alencastro LF, et al. (2015) Effect of advanced oxidation processes on the micropollutants and the effluent organic matter contained in municipal wastewater previously treated by three different secondary methods. Water Res 84: 295-306. [Ref.]
- Ijpelaar GF, Groenendijk M, Hopman R, Kruithof JC (2002) Advanced oxidation technologies for the degradation of pesticides in ground water and surface water. Water Sci Technol: Water Supp 2: 129-138. [Ref.]
- Clarin J, Fletcher M, Reichardt D (2000) UV/ozone/peroxide treatment ENVE. [Ref.]
- Radjenovic J, Matosic M, Mijatovic I, Petrovic M, Barcelo D (2007) Emerging contaminants from industrial and municipal waste: removal technologies, membrane bioreactor (MBR) as an advanced wastewater treatment technology. 37-101. [Ref.]
- Mutamim NSA, Noor ZZ, Hassan MAA, Yuniarto A, Olsson G (2013) Membrane bioreactor: Applications and limitations in treating high strength industrial wastewater. J Chem Eng 225: 109-119. [Ref.]
- Göbel A, McArdell CS, Joss A, Siegrist H, Giger W (2007) Fate of sulfonamides, macrolides, and trimethoprim in different wastewater treatment technologies. Sci Total Environ 372: 361-371. [Ref.]
- Radjenović J, Petrović M, Barceló D (2009) Fate and distribution of pharmaceuticals in wastewater and sewage sludge of the conventional activated sludge (CAS) and advanced membrane bioreactor (MBR) treatment. Water Res43: 831-841. [Ref.]
- Navaratna D, Shu L, Jegatheesan V (2016) Evaluation of herbicide (persistent pollutant) removal mechanisms through hybrid membrane bioreactors. Bioresour Technol 200: 795-803. [Ref.]
- Phan HV, Hai FI, McDonald JA, Khan SJ, van de Merwe JP, et al. (2015). Impact of hazardous events on the removal of nutrients and trace organic contaminants by an anoxic-aerobic membrane bioreactor receiving real wastewater. Bioresour Technol, 192: 192-201. [Ref.]
- Tauseef SM, Abbasi T, Abbasi SA (2013) Energy recovery from wastewaters with high-rate anaerobic digesters. Renewable and Sustainable Energy Reviews 19: 704-741. [Ref.]
- Yang W, Cicek N, Ilg J (2006) State-of-the-art of membrane bioreactors: Worldwide research and commercial applications in North America. J Memb Sci, 270: 201-211. [Ref.]
- Sahar E, David I, Gelman Y, Chikurel H, Aharoni A, et al. (2011) The use of RO to remove emerging micropollutants following CAS/UF or MBR treatment of municipal wastewater. Desalination 273: 142-147. [Ref.]
- Shawaqfeh AT (2010) Removal of Pesticides from Water Using Anaerobic-Aerobic Biological Treatment. Chin J Chem Engineering, 18: 672-680. [Ref.]
- Oh K, Tuovinen OH (1994) Biodegradation of the phenoxy herbicides MCPP and 2,4-D in fixed-film column reactors. Int Bio & Biodeg 33: 93-99. [Ref.]
- GHD (2015) Review of trickling filter technology [Ref.]
- Kaminski W, Kusmierek K, Swiatkowski A (2014) Sorption equilibrium prediction of competitive adsorption of herbicides 2,4-D and MCPA from aqueous solution on activated carbon using ANN. Adsorption 20: 899-904. [Ref.]
- Dickenson ERV, Drewes JE (2010) Quantitative structure property relationships for the adsorption of pharmaceuticals onto activated carbon. Water Sci Technol 62: 2270-2276. [Ref.]
- Grover DP, Zhou JL, Frickers PE, Readman JW (2011) Improved removal of estrogenic and pharmaceutical compounds in sewage effluent by full scale granular activated carbon: Impact on receiving river water. J Hazar Mater 185: 1005-1011. [Ref.]
- Gu L, Chen Z, Sun C, Wei B, Yu X (2010) Photocatalytic degradation of 2, 4-dichlorophenol using granular activated carbon supported TiO2. Desalination 263: 107–112. [Ref.]
- Lenntech (2016) Ozone applications drinking water. [Ref.]
- Parra S, Sarria V, Malato S, Péringer P, Pulgarin C (2000) Photochemical versus coupled photochemical-biological flow system for the treatment of two biorecalcitrant herbicides: Metobromuron and isoproturon. Appl Catal B: Environ 27: 153-168. [Ref.]
- Moreira FC, Vilar VJP, Ferreira ACC, dos Santos FRA, Dezotti M, et al. (2012) Treatment of a pesticide-containing wastewater using combined biological and solar-driven AOPs at pilot scale. J Chem Eng 209: 429-441. [Ref.]
- Vilar VJ, Moreira FC, Ferreira AC, Sousa MA, Gonçalves C, et al. (2012). Biodegradability enhancement of a pesticide-containing bio-treated wastewater using a solar photo-Fenton treatment step followed by a biological oxidation process. Water Res 46: 4599-613. [Ref.]
- Zapata A, Oller I, Sirtori C, Rodríguez A, Sánchez-Pérez JA, et al. (2010) Decontamination of industrial wastewater containing pesticides by combining large-scale homogeneous solar photocatalysis and biological treatment. Chem Engineering J, 160: 447-456. [Ref.]
- Kastanek F, Maleterova Y, Kastanek P (2007) Combination of Advanced Oxidation and/or Reductive Dehalogenation and Biodegradation for the Decontamination of Waters Contaminated with Chlorinated Organic Compounds. Separation Sci Technol 42: 1613-1625. [Ref.]
- Xiong Z, Cheng X, Sun D (2011) Pretreatment of heterocyclic pesticide wastewater using ultrasonic/ozone combined process. J Environ Sci 23: 725-730. [Ref.]
- Oller I, Malato S, Sánchez-Pérez JA, Maldonado MI, Gassó R (2007) Detoxification of wastewater containing five common pesticides by solar AOPs–biological coupled system. Catalysis Today 129: 69-78. [Ref.]
- Sanchis S, Polo AM, Tobajas M, Rodriguez JJ, Mohedano AF, et al. (2013) Degradation of chlorophenoxy herbicides by coupled Fenton and biological oxidation. Chemosphere 93: 115-122. [Ref.]
- Samir R, Essam T, Ragab Y, Hashem A (2015) Enhanced photocatalytic-biological degradation of 2,4 dichlorophenoxyacetic acid. j.bfopcu 53: 77-82. [Ref.]
- Ebrahiem EE, Al-Maghrabi MN, Mobarki AR (2017) Removal of organic pollutants from industrial wastewater by applying photoFenton oxidation technology. Arabian J Chem 10: 1674-S1679. [Ref.]
- Ikehata K, Gamal El-Din M, Snyder SA (2008) Ozonation and Advanced Oxidation Treatment of Emerging Organic Pollutants in Water and Wastewater. Ozone: Sci & Eng 30: 21-26. [Ref.]
- Ikastanek L, Chen Z, Sun C, Wei B, Yu X (2010) Photocatalytic degradation of 2, 4-dichlorophenol using granular activated carbon supported TiO2 . Desalination, 263: 107–112. [Ref.]
- Ahmed MB, Zhou JL, Ngo HH, Guo W, Thomaidis NS, et al. (2017) Progress in the biological and chemical treatment technologies for emerging contaminant removal from wastewater: A critical review. Journal of Hazardous Materials 323: 274-298. [Ref.]
Download Provisional PDF Here
Article Type: Review Article
Citation: Goodwin L, Carra I, Campo P, Soares A (2017) Treatment Options for Reclaiming Wastewater Produced by the Pesticide Industry. Int J Water Wastewater Treat 4(1): doi http://dx.doi. org/10.16966/2381-5299.149
Copyright: © 2017 Goodwin L, et al. This is an open-access article distributed under the terms of the Creative Commons Attribution License, which permits unrestricted use, distribution, and reproduction in any medium, provided the original author and source are credited.
Publication history:
SCI FORSCHEN JOURNALS
All Sci Forschen Journals are Open Access