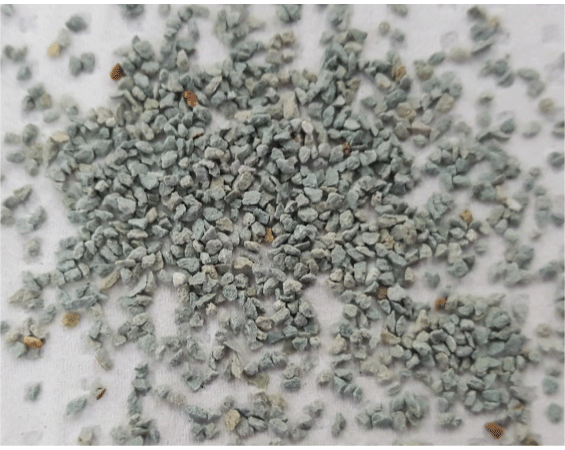
Figure 1: Granular View of the Natural Zeolite Mineral
Amanda L Ciosek* Grace K Luk
Ryerson University, Faculty of Engineering and Architectural Science, Department of Civil Engineering, Toronto, Ontario, M5B2K3, Canada*Corresponding author: Amanda L Ciosek, Ryerson University, Faculty of Engineering and Architectural Science, Department of Civil Engineering, Toronto, Ontario, M5B2K3, Canada, E-mail: amanda.alaica@ryerson.ca
This study investigates the ionic effects of Cu2+, Fe3+, Ni2+ and Zn2+on the sorption of the target metal ion Pb2+ by natural zeolite in the form of clinoptilolite. The batch testing configuration consists of a synthetic nitrate salt solution maintained at 10 meq/L system total concentration, acidified to a pH of 2 by HNO3 acid, with an adsorbent dosage of 40 g/L. The aqueous solution is agitated within a contact period of 5 to 180 minutes by means of a triple-eccentric drive orbital shaker operating at 400 r/min. The objective is to determine the overall sorption of the target metal lead and the selectivity of clinoptilolite to the various heavy metallic ions (HMIs) combined in aqueous phase. Elemental analysis concludes that after 3 contact hours, the lead ion is removed by 76.8% in the single-lead system. In the dual-lead system, Pb2+ is removed by 88.3%, 79.5%, 89.1%, and 88.5% when combined with copper, iron, nickel, and zinc, respectively. Finally, Pb2+ is removed by 90.2% and 94.0% in the triple-(lead-copper-iron) and multi-component systems, respectively. As the HMIs are introduced to the system with a 10 meq/L total concentration, the uptake of the Pb2+ ion in the single-system of 7.68 meq/L is decreased by 75.6% to 1.88 meq/L in the multi-component system. Overall, the total HMIs removed decreases from 7.68 meq/L in the single-system to 3.95 meq/L with the subsequent addition of ions in the multi-component system.
Zeolite; Clinoptilolite; Heavy Metallic Ions; Ion-Exchange; Adsorption; ICP-AES
The Canadian mining industry is thriving in exploration and development, as a global leader in mineral and metal production [1]. This prosperous outlook has been overshadowed by a recent disaster, occurring on August 4, 2014 at Mount Polley Mine of Likely, British Columbia. A total of 25 million cubic meters of contaminated water and mine waste spilled into nearby waterways, from a mine that once extracted copper, gold and silver [2]. This tragedy has disrupted the entire ecosystem and demonstrates the need for further mine discharge treatment development.
Acid mine drainage (AMD) is a primary source of contamination, as waterways are prone to the discharge of mineral mining and processing effluent [3]. Mine operations store their residual drainage in tailings storage facilities (TSF) or ponds, which are profoundly contaminated with heavy metals. These ponds that contain heavy metallic ions (HMI) are extremely dangerous to the peripheral environment. These HMIs are non-biodegradable pollutants [4], which significantly threaten our health with their ability to accumulate in living organisms [4-6]. Based on a census of 30 mines with 75 corresponding stream codes compiled by the Canada-Wide Survey of Acid Mine Drainage Characteristics [7], the annual-seasonal average levels for lead (Pb2+) is 0.50 mg/L, exceeding the mean monthly effluent limit of 0.20 mg/L [8] by 150%. The Occupational Health and Safety Act [9] have identified lead as a designated substance that poses a great risk to our ecosystem, and so it was selected as the target metal in the present research.
The mining industry has developed an interest for exploring sorption as an industrial wastewater treatment method [3,10], based on the demonstrated industrial viability and effectiveness, cost efficiency and environmental sustainability [11,12]. It is important to note that the term sorption refers to every type of capture of a substance as a physical, chemical, and electrostatic process; from the external surface of solids as well as from the internal surfaces of porous solids or liquids. The HMI uptake is attributed to both adsorption (on the surface of the adsorbents’ micropores) and ion-exchange (through the adsorbents’ framework pores and channels) mechanisms [13]. Both are a mass transfer process from a fluid to a solid phase, and the total charge sorbet and desorbed is explicitly the same as required by the electro-neutrality principle. For practical applications, it is generally accepted to collect adsorption and ion-exchange as sorption for a unified treatment process [14,15].
Adsorbent materials investigated include natural zeolites, activated carbon, biomaterials, and clay minerals (i.e. bentonite, kaolin) [16]. Natural zeolites in particular have progressed among researchers’ interests, due to its coexisting molecular sieve action, ion-exchange and catalytic properties [10,17,18]. The mineral zeolite is an alkali and alkaline earth hydro-aluminosilicate [18] crystalline and is a member of the tetkosilicates group [19]. It is composed of a three-dimensional framework built of primary building units (tetrahydra) of silicon-oxygen and aluminumoxygen, which encompass complex rings that create an open homogenous microporous structure of voids and channels, containing cations (Na+, Ca2+, Mg2+, K+) [18]. The ratio of (Al+Si): O is 1:2 and the silicon ions presence is greater than the tetrahedral aluminum ions with a SiO2 :Al2 O3 ratio greater than 2:1. Quadrivalent silicon is replaced by trivalent aluminum, creating a positive charge deficiency to become negatively charged. A balance among charges is achieved through the addition of the mono- and di-valent cations [19]. Clinoptilolite, a globally abundant and well-documented form of zeolite [16,17], is used in this research. As a member of the heulandite zeolite group, clinoptilolite has a monoclinic crystal structure, 39% void volume, as well as an approximate specific gravity and ion-exchange capacity of 2.16 and 2.54 meq/g, respectively [19]. One of the most significant properties of zeolite is its high cation exchange capacity (CEC), which depends on its silica/aluminium ratio [17]. Overall, it is considered as a strong candidate for the removal of wastewater contaminants [20].
The composition of AMD waste contains numerous ions, and the presence of these in solution affect the overall removal [14]. Natural zeolites have demonstrated effectiveness by many researchers, especially on the removal of the target metal lead. However, existing investigations have focused generally on single-ion systems. It is necessary to report on the simultaneous sorption of the multi-metallic components that are prevalent in industrial effluent, and to quantify uptake interference of these HMIs in combination [12]. Therefore, the objective of this research is to investigate the effect of commonly occurring HMIs, such as copper, iron, nickel and zinc, on the overall sorption of the target metal lead. This is to be achieved with a comprehensive experimentation designed to compare the sorption in single, dual, triple, and multi-component systems, to determine the effect of the presence of various HMIs on the selectivity of clinoptilolite in its natural state.
The natural zeolite mineral sample used in this study (as displayed in Figure 1) is composed primarily of clinoptilolite, with traces of opaline silica, and is sourced by a deposit located in Preston, Idaho [21]. The composition and relevant mineralogical specifications of this sample are summarized in Table 1. The general chemical formula of the zeolite sample is expressed as (Na,K,Ca)(2-3)Al2(Al,Si)2 Si13O•12H2 O. No significant concentrations of toxic trace elements are present in its composition, nor are trace metal elements water soluble. Also, no crystalline silica by XRD analysis is detected. The low-clay content unique to this sample ensures no clouding in water and low dust content, with a harder and more resistant structure [21]. In order to reduce the overall cost and associated environmental impacts of the process, the zeolite mineral is applied in its natural state; with no chemical modifications. The particle size distribution of the mineral is obtained from standard mesh sieves and a mechanical shaker, and arrange of sizes of 0.841 to1.19 mm is used for the experimentation. The particles are put through a cleaning cycle, which involves rinsing in deionized distilled water to remove residual debris and dust, and drying at 80 ± 3°C for 24 hours to remove residual moisture [22].
Figure 1: Granular View of the Natural Zeolite Mineral
Table 1:Natural Zeolite Specifications [21]
The Canada-Wide Survey of Acid Mine Drainage [7] summarizes data from various mine types such as copper-zinc and nickel-copper. The survey also isolates waste from different sources that include mine water, tailings pond water, seepage/runoff/streams, tailings effluent, and treatment/collection systems that can be found across Canada. Based on metal processing mines at the time of this survey, the metals predominately found in AMD streams are lead, copper, iron, nickel, zinc, arsenic, cadmium, cobalt, aluminum, and manganese. The Canadian Minister of Justice Metal Mining Effluent Regulations outline the authorized limits of deleterious substances (Schedule 4) [8], for arsenic, copper, cyanide, lead, nickel, and zinc. This regulation also highlights the need for effluent characterization water quality monitoring based on the concentrations of aluminum, cadmium, iron, mercury, selenium, ammonia, and nitrate. Due to a greater presence in Ontario mines presented by Wilson [7] and the strict limitations required by the Canadian Government [8], this study focuses on the presence of lead, in combination with copper, iron, nickel and zinc [23]. Several reports have investigated both batch and column configurations in primarily single-component systems [24]. It is important to highlight that existing literature is limited in how these five specific heavy metals interact in various systems (single, dual, triple, and multiple) with zeolite in its natural, untreated state. This study provides major insight into this uncertainty, with a focus on the heavy metal lead.
The cations present in the adsorbent have valences that differ from those in solution. Consequently, as the dilution increases, the selectivity of the adsorbent for the ion with a higher valence also increases. Accordingly, comparative analysis of various metal ions should be conducted at the same normality and temperature, in order to minimize the changes observed in isotherm configuration with dilution [25]. The synthetic metallic ion solutions are prepared from analytical grade nitrate salts, namely Pb(NO3 )2 , Cu(NO3 )2 •3H2 O, Fe(NO3 )3 •9H2 O, Ni(NO3 )2 •6H2 O, and Zn(NO3 )2 •6H2 O, based on a total normality of 0.01N (10 meq/L) as investigated by Inglezakis et al. [24] and Ciosek and Luk [26] for meaningful comparisons. With lead as the targeted metal, the metals are combined to maintain an overall 10 meq/L (0.01 N) normality concentration, in the systems that follow. It is important to note that this study systematically increases the HMI combinations to develop a more comprehensive understanding of how the presence of multiple HMIs influences the removal of lead.
The seasonal average of a majority of the mines surveyed by Wilson [7] documented pH values ranging from 2 to 5. The acidity of the batch solution during the ion-exchange process is paramount, with the H+ ion initially present considered in competition with the targeted HMI. Consequently, the influent stock is acidified with concentrated nitric acid to a pH level of less than 2 [27] to prevent precipitation of the metal ions [10,25].
Batch sorption tests are conducted by mixing synthetic heavy metallic ion (HMI) solutions with an adsorbent dosage of 40 g/L. The mixture is agitated on a bench-top orbital shaker with triple-eccentric drive at 400 r/mins at 22°C, for a contact period ranging from 5 to 180 minutes. In all experiments, after reaction, solution and adsorbent were separated through a 0.45 μm filter syringe. The HMI concentrations are analyzed in their aqueous phase with Inductively Coupled Plasma – Atomic Emission Spectroscopy (ICP-AES) technology. The calibration curve is generated through linear calculated intercept by applying a stock blank and a multi-element Quality Control Standard 4 with 1,10,50,90, and 100 mg/L concentrations. The adsorbed amount of HMI was calculated from the difference between the starting concentration and its concentration in the supernatant. The zeolite is analyzed further to observe the surface topography with HMI exposure in the multi-component system over time, through Scanning Electron Microscopy (SEM) technology.
Table 2 provides a summary of the sample preparations involved for elemental analysis. The ‘Sample ID’ column outlines each component system [Single, Dual-D, Triple-T, and Multi-M] and their respective influent stock (S) and contact times, which range from 5 to 180 minutes. The number of 50% dilutions are indicated in the column ‘DIL’, which is required to keep all concentrations within the calibration range of 0-100 mg/L. Based on the established adsorbent-to-adsorbate ratio of 4 g to 100 mL, the measured ‘Zeolite Mass’ is provided for each sample. Finally, the ‘pH Level’ verification results are provided for both directly from sample on the day of zeolite exposure, and the day after of the filtered sample.
Table 2: Sample Preparation with pH Level Verification
It is important to note that the acidity of the adsorbate solution affects the uptake of metals and its speciation [28], and this is particularly the case for HMIs that are in rather low preference by zeolite. Consequently, the hydrogen cation (H+) is considered as a competitive ion to the HMI during the ion-exchange process. During this process, the uptake is favoured by high acidity (low pH level). This pH level should be lower than the minimum acidity of precipitation for each metal of interest. Presented in Equation 1 [25] is the two-way reversible relationship between the hydrolysis and hydration of metals, which is affected by the acidity of the solution [25].
[M(H2O)]n+ + H2 «[M(H2 O)x-1(OH)](n-1) + H3 O+ (1)
At a lower pH level, the reaction is shifted to the left and more highly charged metal complexes are formed with inorganic ligands such as OH- [28], which encourages the adsorption and ion-exchange processes. However, this condition is not favourable as the H+ cations compete with the HMI in solution, adversely affecting the desired outcome [25]. The degree of metal complex formation depends on the pH level, ionic composition and HMI of interest. Also, the metal speciation influences the removal efficiency of the adsorbent and the adsorbent’s affinity to a given metal is affected by the metal complex characteristics that predominates at a given pH level [28].
Research conducted by Ersoy and Celik [20] investigates the zeta potential of clinoptilolite, and demonstrates its electro-kinetic properties (i.e. is electric point, potential determining point) in aqueous solutions, which are critical in the understanding of adsorption mechanisms of the solid-solution interface. Isomorphic substitutions of the Si4+ and Al3+ ions in its crystal structure with exchangeable cations (i.e. Na+, Ca2+, Mg2+, K+ include the hydrogen ion to account for the surface charge. Consequently, the pH level of the system is predicted to affect the sorption behaviour. The potential determining ions were verified to be H+ and OHfor metallic cations of interest. The isoelectric point refers to when the sum of all interactions (i.e. H+ and OH- adsorption, dissolved lattice ions distribution, etc.) occurring at the mineral-water interface has a net zero charge. Ersoy and Celik [20] demonstrated that at a pH level of 2, the zeta potential of the clinoptilolite surface is approximately −18 mV; showing a permanent net negative charge at very acidic conditions, which provides an advantage during electrostatic interactions of inorganic cationic contaminants in wastewater.
The natural acidity and stability constants of metal complexes are significant. The natural acidities have been reported as 4 to 3 for Pb2+ and Cu2+, and 2 for that of Fe3+. The initial pH level of the solution must be selected attentively, ensuring balance among all ionic species involved. The aim is to avoid precipitation, for once precipitated; the ions of interest cannot be sorbet. Also, the structural stability of the adsorbent should not be compromised. For example, the structure of clinoptilolite breaks down at pH levels below 1 in a process termed ‘dealumination’ [25]. Furthermore, in highly acidic solutions, the hydrogen cation (H+) would be a highly competitive ion to the HMI of interest. With this in mind, the prepared influent stock was acidified to a level of 2. Following adsorbateadsorbent contact, the pH is observed to be steady throughout the ionexchange process, with a maximum of 0.20 pH level difference after 3 contact hours. The pH levels of the prepared influent stock and filtered effluent are also observed to be consistent during refrigerated storage prior to dilution preparation for elemental analysis.
The Inductively Coupled Plasma – Atomic Emission Spectroscopy (ICPAES) technology (Optima 7300, Perkin Elmer Inc., USA) was employed in radial view with corresponding WinLab32 Software. The quantitative results of the various systems are summarized in Table 3. The primary wavelengths, given in Column 1, to the ICP-AES model were applied for all five metal ions to generate the Relative Standard Deviation (%RSD) as shown in Column 3. Triplicate readings and their mean concentrations in calibration units are presented in mg/L, and given in Column 4. Based on the dilution factors presented in Table 2, the corresponding HMI concentrations and percent removal after 3 hours were calculated and provided in Column 5 and 6, respectively. Overall, the %RSD values are low and all within <3%, which is indicative that the data is accurate and the experimental replicates are reliable [29]. A significant observation is made for that of the target metal ion. The overall removal of the lead ion is highest compared to the four other HMI investigated. In addition, this removal level is consistently high regardless of the component system. This is a first glimpse in this study of zeolite’s affinity to that of lead, and the overall impact of the presence of the other ions.
Table 3: ICP-AES HMI Results of Various Component Systems
Figure 2 exhibits SEM images of natural zeolite, which were obtained by a high-resolution microscope (6380LV, JEOL, USA), equipped with Oxford energy dispersive X-ray spectroscopy (EDS) and electron backscatter diffraction (EBSD) capacity. The images taken at 1000X magnification (10 µm scale bar) illustrate the physical changes of the surface structure comparing the raw granules (shown in 2a) and those exhibited to the cleaning cycle of debris and moisture removal (shown in 2b). The images of the zeolite exposed to the multi-component solution containing all metals over the 3 and 6 hours of contact are provided in (2c) and (2d), respectively. Once the residual debris and moisture are removed, it is observed that the zeolite structural complexity has been prepared for HMI sorption; with visual evidence of textured granularity and significant detail relative to the images of M180 and M360. The inter-granular spaces and mineral crevasses have been subsequently smoothed out over time, giving a visual indication that the surface structure of the material has been filled with and occupied by the ionic metals. This provides additional insights into how the HMIs are removed on the surfaces of the zeolite particles.
Figure 2: SEM Images of Natural Zeolite Exposure to Multi-Component Solution Over Time
Several measures were applied to ensure quality control of the experimental investigations carried out in this study. During the preliminary stages of investigation, all of the batch sorption experiments were conducted twice. Filter separation was investigated with 5 and 180 minute effluent samples, with a first then second filter pass. Only a maximum of 1.5% difference among the filtered sets was measured, and supports that the agitation method in triple-eccentric drive for adsorbentadsorbate contact is effective. These preliminary findings demonstrated that the protocol established is repeatable and moving forward, all other tests were run once per sample. Furthermore, each sample concentration is read in triplicates as set in the ICP-AES system software for validation purposes. Quality control checks were applied to both the calibration standards and the sorption results. Table 4 summarizes the QC checks conducted on the calibration standard of 50 mg/L for the various component systems. The %RSD values are considerably lower than the 3% targeted limit, and a majority of detected concentrations fall well within 5% of the 50 mg/L theoretical value.
Table 4: Calibration Standard 50 QC Check of Various Component Systems
Figure 3 displays the percent removal trend of lead over time, and how it varies among the various metallic heavy metallic ion (HMI) component systems. The elemental analysis results have indicated that after just 3 hours of contact with natural zeolite, the target metal ion lead is removed by 76.8% in the single-lead system. In the dual-lead system, the lead ion is removed by 88.3%, 79.5%, 89.1%, and 88.5% when combined with copper [DCu], iron [DFe], nickel [DNi], and zinc [DZn], respectively. The targeted lead ion is removed by 90.2% and 94.0% in the triple-(lead-copper-iron) [T], and multi-component [M] systems, respectively. In the multicomponent system involving all five metals, thepercent removal observed for Pb2+, Cu2+, Fe3+, Ni2+, and Zn2+ are 94.0%, 21.9%, 56.2%, 9.10%, and 16.5%, respectively. The corresponding removal trends are illustrated in Figure 4. Zeolite’s affinity towards each metal ion is also observed in the triple-component system, with a percent removal of 90.2%, 19.0%, and 49.5% for that of Pb2+, Cu2+, and Fe3+, respectively.
Figure 3: Percent Removal of Lead in the Various Component Systems
Figure 4: Percent Removal of All HMIs in the Multi-Component System
Additional observations are revealed when the percent removal of each HMI of the various component systems is investigated further. With Pb2+ as the ion of interest in this study, Table 5 shows that only 1.88 meq/L out of a possible 2.0meq/L in the multi-component system versus 7.68 meq/L of 10 meq/L in the single-component system. Zeolite’s highest affinity is for that of lead, and is followed by iron. In the dual-iron [DFe] system, 3.97 meq/L of 5.0 meq/L is removed and in the triple [T] system, 3.01 meq/L of 3.3 meq/L is removed once copper is introduced.
Table 5: The HMI Removal (meq/L) in the Various Component Systems Relative to the Lead Ion
This removal efficiency order is indicative of how the adsorptive nature of zeolite for each ion varies among the different component systems. Based on the adsorbent-to-adsorbate ratio of 4 g to 100 mL, the HMI uptake is calculated by Equation 2 [11,12,25] and is summarized in Table 6:
Table 6: The HMI Uptake in the Various Component Systems by Zeolite
\[Q\left( t \right) = \frac{{V \times \left( {{C_0} - {C_t}} \right)}}{M}\,\,\,\,\,\,\,\,\,\,\,\,\,\,\,\,\,(2)\]
Where Q(t) is the HMI adsorbed at time (in meq/g), and are the initial and final HMI concentrations in solution (in meq/L) after time, is the solution volume (in L), and is the zeolite mass (in g). For a HMI pair evaluated as A/B, the selectivity ratio (SR) is determined by Equation 3 and presented in Table 7, where is the uptake at time (in meq/g) [25]:
Table 7: Selectivity Ratios (SR) of the Various Component Systems Relative to Lead Uptake
\[SR = \frac{{{Q_A}\left( t \right)}}{{{Q_B}\left( t \right)}}\,\,\,\,\,\,\,\,\,\,\,\,\,\,\,\,\,\,\,\,\,\,(3)\]
As HMIs are introduced to the system, the overall uptake of the lead ion and the total HMIs are decreased. An uptake of lead ions by zeolite is reduced by approximately 75.6%, going from 0.1919 meq/g in the single-component system to 0.0469 meq/g in the multi-component [M] system. Also, in the presence of highly competitive ions, the removal of Pb2+ is hindered. This is indicative of zeolite’s selectivity, where the Pb/ Fe ratio is the lowest and the Pb/Ni as the highest, with a greater uptake of lead in combination of nickel than with iron. Furthermore, copper is seen as the third ion of appeal to that of zeolite. When introduced in the triple-component system, it is observed that the overall uptake of HMIs is reduced from 0.1462 meq/g in the [DFe] system to 0.1320 meq/g in the [T] system. These key trends are indicative of the selectivity of zeolite for the various HMIs in solution.
Figure 5 represents the total uptake of HMI in the various component systems over the 3 hour analysis period. It is evident that equilibrium has yet to be reached based on the established experimental conditions; however, a curve-to-plateau is detected and can be indicative of the longterm trends. As demonstrated by Motsi et al. [30], the initial stage of rapid adsorption occurs within the first 40 minutes of contact. This is when all of the adsorption sites are available for cation interaction to occur, and when the concentration difference between the influent stock and adsorbentsolution interface is very high. Inglezakis et al. [24] credits this period to ion-exchange in the micro-pores on the zeolite particles’ surface. Slower adsorption follows, which is attributed to slower diffusion of the cations in solution into the internal network of the zeolite, such that these cations must occupy the exchangeable sites within the channelled structure [30].
Figure 5: Total HMI Uptake in the Various Component Systems over Time
The presence of each HMI in solution impacts the uptake of lead; however, the removal efficiency trend is maintained throughout the various component systems. It is observed that the adsorbent mineral zeolite has a favoured uptake for that of the Pb2+ ion, followed by Fe3+ and Cu2+, with a lower affinity to Zn2+ then Ni2+. The selectivity study conducted by Anari-Anaraki and Nezamzadeh-Ejhieh [11] involved surface surfactant modified clinoptilolite nanoparticles, with Pb2+ ion dual-component systems and interfering cations; supporting reported literature trends as: Pb2+>Cd2+>Cs+>Cu2+> Co2+>Cr3+>Zn2+>Ni2+. This selectivity series is also consistent with the results obtained by Inglezakis et al. [25], which investigated the trends of lead, copper, iron and chromium ions in various component systems as well as the findings of Ouki and Kavannagh [28,31], which studied the selectivity and removal performance of lead, copper, cadmium, zinc, chromium, cobalt and nickel in a mixed system. Their research has determined that both physicochemical and stereochemical factors (i.e. micropore spacing constraints, hydrated radii (ion-to-pore ratio), cation hydration enthalpy) influence the removal efficiency.
Ouki and Kavannagh [31] emphasize that clinoptilolite is comprised of a: (1) high silica/aluminium ratio, (2) low volumetric capacity, and (3) weak ionic field, within the structure. Therefore, the cation hydration free energy (a measure of water molecule rejection) [25] is considered more significant than the electrostatic interactions within the framework during the sorption process. HMIs with higher hydration energy prefer to remain in solution, where their hydration conditions are well-fulfilled. The hydration energy of Pb2+ (−357 kcal/mol) compared to Cu2+ (−502 kcal/ mol) and Fe3+ (−1053 kcal/mol) suggests that lead is superior in uptake to the other ions as it is not composed of complex, hydrated compounds and thereby demonstrates the significantly higher selectivity of lead by clinoptilolite [25,31]; this general observation further substantiated by the contribution of this present study.
The work of Helfferich [14] has highlighted that mobile species (counter ions, co-ions, and neutral particles) and various factors influence sorption equilibria, which affects the affinity of an ion-exchanger for a given ion in multi-component electrochemical systems. Specifically for the lead ion of interest to this study, the factor that anion-exchanger prefers the counter-ion which associates less strongly (participates least in complex formation) with the co-ion is of importance. This finding is concluded by Inglezakis et al. [32]; discussing the formation of the various hydrated ions of the iron, copper, and chromium investigated and their corresponding stable species. However, the Pb2+ studied typically does not have a strong tendency to form strong complexes, and gives reasoning for its preferential uptake by zeolite.
In practical applications of AMD waste, the presence of multiple HMIs in solution affects the overall treatment process. This study has demonstrated that the natural zeolite clinoptilolite is effective in the uptake of the target metal lead. Finally, further insights and quantitative details have been presented for using the sorption process as a valuable asset to wastewater treatment.
This study investigated the predominant heavy metallic ions found in mine waste streams, and how in combination, the ionic removal of lead is affected with their presence and varies over time. The significant findings from this research are as follows:
The discoveries presented are critical to both the mining and environmental industry, which advance the design of treatment systems through experimentation, modelling, and simulation. This removal method may be a predictive indicator for the removal of other metallic ions from industrial effluent. This research achieves a deeper insight into how the presence of multiple metallic ions impacts the overall removal efficiency of the target metal lead. The data obtained is highly useful as the basis for designing future experimental studies in equilibrium isotherms, reaction kinetics, as well as sorption simulations.
This research was conducted with the financial support of an NSERC Discovery Grant to Grace K. Luk. We would like to thank Dan Mathers, the Supervisor of the Analytical Lab for Environmental Science Research and Training (ANALEST) at the University of Toronto at the time of analysis, for providing insight and expertise that greatly assisted this study.
Download Provisional PDF Here
Article Type: Research Article
Citation: Ciosek AL, Luk GK (2017) Lead Removal from Mine Tailings with Multiple Metallic Ions. Int J Water Wastewater Treat 3(1): doi http://dx.doi. org/10.16966/2381-5299.134
Copyright: © 2017 Ciosek AL, et al. This is an open-access article distributed under the terms of the Creative Commons Attribution License, which permits unrestricted use, distribution, and reproduction in any medium, provided the original author and source are credited.
Publication history:
All Sci Forschen Journals are Open Access