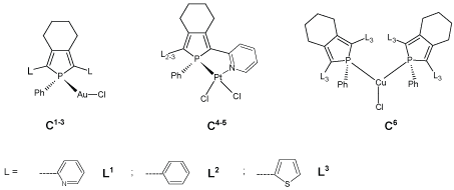
Figure 1: Metal-phosphole complexes (C1-6).
Samuel Alfonso-Bueno1,2 Peter Taylor2 Izaskun Urdanibia2 William Castro3* Yomaira Otero3*
1Universidad de los Andes, Facultad de Ciencias, Departamento de Química, Mérida, Venezuela*Corresponding author: Otero Y, Centro de Química, Instituto Venezolano de Investigaciones Científicas, 1020-A, Caracas, Venezuela, Tel: +58 212 5041637; E-mail: yotero@ivic.gob.ve; yomaira@gmail.com
Castro W, Centro de Química, Instituto Venezolano de Investigaciones Científicas, 1020-A, Caracas, Venezuela, Tel: +58 212 5041642; E-mail: wcastro@ivic.gob.ve; wcastro10@gmail.com
It is well-known that Au(I) complexes are potentially attractive as anticancer agents due to their strong antiproliferative potency and inhibit the enzyme thioredoxin reductase (TrxR) with high potency and specificity. Au(I) phosphine complexes have shown great potential as anticancer agents, however, their efficacy has been limited by their high toxicity and lack of selectivity for cancer cells. The design of an effective anticancer agent is a complicated game, thus the study of new coordination complexes for cancer treatment is necessary. In this work, we have synthesized Au(I)-Phosphole complexes (C1 , C2 , and C3 ) using different π-conjugated phospholes derivatives such as: 2,5-bis(2-pyridyl)-1-phenylphosphole (L1 ), 1,2,5-triphenylphosphole (L2 ) and 2,5-bis(2-thienyl)-1-phenylphosphole (L3 ) respectively and evaluated their interaction with DNA, as the main target of action of anticancer drugs through spectroscopic titration, viscosity, distribution coefficient, electrophoresis and inhibition of tumor cell lines. We have observed that Au(I)-phosphole complexes (C1-3) caused a decrease in the relative viscosity of DNA compared to the free phosphole derivatives probably due to bends or kinks in the DNA helix. The electrophoresis and spectroscopic titration results suggested that all the compounds bind to CT-DNA through weaker interaction (noncovalent). In addition, we have found that compounds C1 and C2 have no effect on the growth or viability of the cells while C3 inhibited the cell growth of human breast carcinoma (MCF7) and human prostate carcinoma (PC3) cancer cells in vitro at concentrations below 30 µM and in this way corroborated the relationship between the study of interaction with DNA and cytotoxic activity.
Au-Phosphole complexes; π-Conjugated Phospholes; DNA; Thioredoxin; Cytotoxicity
Metal complexes have gained considerable interest in both the development and the treatment of cancer. For example, platinum compounds are well established in current cancer [1]. Cisplatin is one of the most widely used metal-based antitumor drugs targeting DNA. Although it is active in the treatment of several types of cancers, the side-effects limit its potential efficacy [2,3]. Consequently, there is a considerable need for the development of novel metallo drugs and treatment alternatives. Among the new non-platinum drugs especially gold complexes have been focus of attention due to their strong tumor cell growth inhibiting effects, showing unique biological and medicinal properties [4,5]. Particularly, Au(I) phosphine complexes have shown great potential as anticancer agents, however, their efficacy has been limited by their high toxicity and lack of selectivity for cancer cells [6].
On the other hand, the phospholes offer a wide versatility of coordination modes and reactivity patterns [7]. Structural diversity of their transition metal complexes has been facilitated by the ability of the phosphorus ring to coordinate metals in σ, π, and mixed bonding modes [8,9]. Phosphole complexes have been used in fields as diverse as catalysis, OLEDs, WOLEDs and non linear optics [8,10-17]. Modification of the nucleophilic phosphorus atom by coordination to metal centers permit fine-tuning of the electronic properties (HOMO and LUMO levels, effective conjugation length, etc.) of phosphole-based π-conjugated systems [14,18]. This is important in medicinal inorganic chemistry as it permits modulation of the biological activity (increased affinity and/or reduction of side effects) of such complexes against specific targets, such as DNA or human disulphide reductases, which may render these compounds promising candidates for chemotherapeutic applications [1,4,19].
Gold(I)– and platinum(II)–phosphole complexes (C1-5, Figure 1) have shown to be potent Thioredoxin reductase (TrxR) inhibitors, with the gold(I) complexes being the best GR (glutathione reductase) inhibitors. Their inhibitory properties are complemented by a high affinity for DNA, resulting in toxicity towards glioblastoma cells [20]. The inhibition of human GR by the complex [Au{1-phenyl-2,5-bis(2-pyridyl) phosphole}Cl] (C1 ) is associated with many cellular processes such as antioxidant defense, redox balance, regulation of various proteins, and nucleotide metabolism [21, 22].
Figure 1: Metal-phosphole complexes (C1-6).
Recently, we have evaluated the biological activity of [Cu{1- phenyl-2,5-bis(2-thienyl)phosphole}2 Cl] (C6) in terms of binding to calf thymus (CT) DNA, distribution coefficient, interaction with TrxR and its cytostatic and cytotoxic activities, particularly against the PC3 prostate inhibition of tumor cell lines. We found a noncovalent interaction with DNA, a weak interaction with CT-DNA and that the TrxR system does not seem to be a target of action. Additionally, we studied the lipophilicity of the compound C6 , finding that it may penetrate the cell membrane more effectively than the ligand or cisplatin, this compound showed little inhibitory effect on tumor cell lines [23].
The design of an effective anticancer agent must include not only the drug’s inherent inhibitory properties but also its delivery, dosage, and residence time in vivo, such that both the selection of metal and the design of ligands are considered important prerequisite for the construction of a highly efficient metal-based drug. With these considerations in mind, it may be fruitful to expand research into Au(I)-phosphole complexes. Thus, we studied the interaction of different Au(I)- phosphole complexes (C1-3, Figure 1) with DNA, as well as their antiproliferative action against cancer cell lines.
All reactions were carried out under an Argon atmosphere using standard Schlenk techniques, unless otherwise stated. The solvents were previously dried and distilled by standard techniques [24].
Synthesis of 1-phenyl-2,5-bis(2-pyridyl)phosphole (L1), 1,2,5-triphenylphosphole (L2) and 1-phenyl-2,5-bis(2-thienyl) phosphole (L3)-To a tetrahydrofuran solution (40 mL) of corresponding octa-1,7-diyne (0.83 mmol) and Cp2 ZrCl2 (0.24 g, 0.83 mmol) was added dropwise, at –78°C, a hexane solution of 1.6 M n-BuLi (1.06 mL, 1.7 mmol). The reaction mixture was warmed to room temperature and stirred for 12 h. To this mixture, solution was added, at –78°C, freshly distilled PhPBr2 (0.250 g, 0.91 mmol). The solution was allowed to warm to room temperature and stirred for 18 h. The solution was filtered on basic alumina under inert atmosphere and the volatile materials were removed in vacuo. The product was washed with freshly distilled pentane (10 mL) and was obtained as a solid [14,25].
Synthesis of [Au{1-phenyl-2,5-bis(2-pyridyl)phosphole}Cl] (C1), [Au{1,2,5-triphenylphosphole}Cl] (C2) and [Au{1-phenyl2,5-bis(2-thienyl)phosphole}Cl] (C3)-A solution of [AuCl(THT) (0.175 g, 0.55 mmol) and the corresponding phosphole (0.55 mmol) in CH2 Cl2 (20 mL) was stirred for 1h at room temperature under argon. Then all the volatile materials were removed under vacuum. The residue was washed with pentane (3 x 10 mL) and dried at 40°C under vacuum [26,27].
Viscosity measurements-All experiments were carried out using an Ostwald viscometer immersed in a water bath maintained at 25 °C. The DNA concentration (65 μM, calf thymus DNA in 5 mM Tris–HCl, 50 mM NaCl and pH 7.54) was kept constant in all samples, while the molar ratio between [compound] and [DNA] was changed from 0 to 1, increasing the concentration of the compound in solution from 0 to 67.5 μM. The mean flow time was measured four times. Data are presented as (η/η0)1/3 versus the ratio [compound]/[DNA], where η and η0 are the specific viscosity of DNA in the presence and absence of the compound, respectively. The values of η and η0 were calculated from the expressions η=t – ts and η0 =t0 – ts , where t is the observed flow time of the DNA-compound, ts is the flow time of DMSO-buffer (using 10% DMSO) and to is the flow time of DNA alone. The relative viscosity of the DNA was calculated from η/η0 [28], using ethidium bromide as a classical intercalator control.
Electrophoresis-For the DNA electrophoresis assays, 10 μL samples of the pBR322 plasmid (20 μg/mL) were combined with the compounds L1-3 and C1-3 at different ratios (molar ratios of compound/DNA (Ri) 1-4) and then incubated for 18 h at 37°C. The reaction was then quenched by the addition of NaCl (1 M) to give a final chloride concentration of 0.2 M. Each sample (5 mL) was run (100 V for 40 min.) on a 0.7% agarose gel with TBE 1X (0.45 M Tris-HCl, 0.45 M boric acid, 10 mM EDTA) and stained with ethidium bromide [29]. The bands were then viewed with a transilluminator and the image captured using a camera Cool Snap HQ2 (BIO-RAD), Universal HOOD III model [30].
Spectrophotometric titrations-Absorption titration experiments were carried out by stepwise additions of a CTDNA solution (1.38 mM, in 5 mM Tris–HCl, pH 7.54 and 50 mM NaCl buffer) to a solution of each compound (0.4 μM) in DMSO, recording the UV–vis spectra after each addition. Native DNA absorption was subtracted by adding the same amounts of CT-DNA to the blank cell. The binding affinity (Kb) was calculated from the spectrophotometric data according to the equation [31].
\[\frac{{[DNA]}}{{{\varepsilon _a} - {\varepsilon _f}}} = \frac{{[DNA]}}{{{\varepsilon _0} - {\varepsilon _f}}} + \frac{1}{{Kb({\varepsilon _0} - {\varepsilon _f})}}\]
Where [DNA] is the concentration of DNA in base pairs, εa is the extinction coefficient of the observed absorption band at the given DNA concentration (corresponding to Aobs/[compound]), εf is the extinction coefficient of the free compound in solution, and εb is the extinction coefficient of the compound when fully bound to DNA. A plot of [DNA]/[εa – εf ] versus [DNA] gave a slope 1/[ εa – εf] and a Y intercept equal to 1/Kb[εb – εf]. Kb is the ratio of the slope to the intercept.
Distribution coefficient (D- The water/n-octanol distribution coefficients were determined by the stir-flask method [32, 33]. UV-visible (UV-vis) calibration curve for each compound in n-octanol-saturated water in the 2–50 μM range was prepared. A mixture of 5 mL n-octanol and 5 mL water (Tris-HCl 5 mM; NaCl 50 mM; pH 7.43), each saturated in the other, was stirred for 30 min. at room temperature, after adding the sample to be analysed. Once equilibrium was reached, the organic and aqueous phases were separated and an aliquot of n-octanol was taken to determine spectrophotometrically the concentration of the compound in each phase. Experiments were carried out by triplicate. Log P, the distribution constant, was calculated from Log P=Log [compound in n-octanol]/[compound in water] [34].
Growth inhibition and cytotoxicity-Five human and one murine tumour cell lines were used. MCF7 (human breast carcinoma), PC-3 (human prostate carcinoma), A459 (human lung carcinoma), HeLa (human cervical carcinoma), HT-29 (human colon carcinoma), and 4T1 (murine breast carcinoma) cells (ATCC American Type Culture Collection, USA) were cultured in Dulbecco’s Modified Eagle’s Medium (DMEM) supplemented with 10% heat-inactivated foetal bovine, (Gibco, BRL, USA) and penicillin (100 Units/mL) / streptomycin (100 μg/mL), containing, in addition, glucose 0.45% for the HT-29 cells. The sulphorhodamine B (SRB) assay was used to evaluate the effect of the compounds on the growth and viability of the six tumour cell lines [35]. This assay is based on the binding of negatively charged sulphorhodamine to basic amine acids in the cell, thus enabling an estimate of cell mass. Each drug was dissolved in DMSO then assayed in triplicate at seven different concentrations (0.1-30 μM), in 3 independent experiments. Internal control wells were included to ensure that the highest concentration of DMSO used did not affect the cells. The concentrations inducing 50% growth inhibition (GI50), total growth inhibition (TGI) and 50% cytotoxicity (LC50) after a 48 hr incubation period were calculated by linear interpolation from the adjacent data points.
Viscosity measurements-In order to establish the mode of compounds/DNA interactions, we carried out viscosity experiments, as it is often used as the least ambiguous and easy method to clarify the interaction between the DNA biomolecule and the compound of interest [36,37]. Variations in the viscosity of DNA are unequivocally associated with changes in its length and are very sensitive to drug intercalation or non-intercalation binding [38]. For example, ethidium bromide, a well-known DNA intercalator increases the relative specific viscosity of DNA due to the lengthening of the DNA double helix resultant from intercalation [39]. Here we observed slight decrease in the relative viscosity of the DNA in the presence of the phosphole derivatives (L1-3) (Figure 2) compared to the results obtained by the EtBr, suggesting bends or kinks in the DNA, or no strong interaction with the DNA, this behavior has been evidenced for similar compounds, and it is due to the size and the and non-planarity of the phosphole molecule that prevents it from being intercalated in the DNA. On the other hand, Au(I)- phosphole complexes (C1-3) showed a similar behavior causing a slight decrease in the relative viscosity of the DNA (Figure 2), this behavior is justified because the coordination of the metal center to the phospholes does not produce significant changes to the structure of the ligand, and do not intercalate the macromolecule. Thus, we suggest that the complexes (C1-3) no significantly change the three-dimensional structure of DNA, as have been found for complexes of Pt (II), Au (III) and Ag (I) which did not present an intercalation interaction [40-42]. However, this does not mean that they can have another type of interaction with DNA.
Figure 2: Effect of increasing concentration of the compounds on the relative viscosity of CT-DNA at 25°C. Relative viscosity (η/η0)1/3 vs [Compound]/[DNA].
Electrophoresis-Gel electrophoresis experiments using pBR322 DNA were performed with compounds L1-3 and C1-3. Agarose gel electrophoresis is a rapid and sensitive method, which shows changes in pBR322 DNA mobility when this molecule interacts with a compound. This plasmid has two native forms, supercoiled (Form I) and circular (Form Io). Drug interaction may cleave the plasmid, resulting in the cut (Form II) or linear form (Form III) and, in extreme cases, produce fragmentation. These cleavage events change the migration profiles on agarose gels. Transition metal complexes have been reported to inhibit DNA repair enzymes [43,44]. Figure 3 shows the migration of the plasmid after incubation with the compounds at different molar ratios (Ri). The addition of the free phosphole ligands did not lead to changes in the circular and supercoiled forms, so that there is little or no interaction with the DNA [45,46], while with the C1-3 complexes, a degradation in the band corresponding to the circular shape is observed (Ri=4), which can be attributed to interaction with the DNA helix [47]. When comparing these results with those obtained for cisplatin, a reference drug, a weaker interaction between the C1-3 complexes and the DNA is evident.
Figure 3: Agarose gel showing the cleavage of pBR322 DNA incubated for 18h at 37°C, with different concentrations of the compounds. Lane 1: Ri=1, Lane 2: Ri=2, Lane 3: Ri=3, Lane 4: Ri=4, where Ri=[compound]/[DNA] molar ratio.
Spectrophotometric titrations-The binding of intercalative drugs to the DNA helix has been characterized classically through absorption spectral titrations, as a function of added DNA [42]. An easy way to determine whether there is any interaction between the DNA and the drug is therefore to examine band shifting of the compound [48]. A complex binding to DNA through intercalation usually results in hypochromism and bathochromism, due to a strong stacking interaction between an aromatic chromophore and the base pairs of DNA [42]. Absorption spectra of compound C3 in presence of DNA are shown in Figure 4. In general, the absorptions bands of all the compounds were affected with increasing concentrations of DNA, with a tendency towards hyprochromism, indicating that the compounds bind to CT-DNA through weaker interaction (non-covalent). In addition, Kb values (Table 1) indicate that only the complex C1 shows higher value binding interaction with the macromolecule [49,50].
Figure 4: Spectroscopic titration spectrum of [Au{1-phenyl-2,5-bis(2- thienyl)phosphole}Cl] (C3) with CT-DNA.
Compound | Absorbance (±1 nm) | Δλ (±1 nm) | Kbx104 (M-1) | Hyprochromism (%) |
L1 | 369 | 21 | 3.70±0,58 | 39±3 |
C1 | 390 | 11.94±0,97 | 11±2 | |
L2 | 369 | 0 | 2.46±0,58 | 23±2 |
C2 | 369 | 2.81±0,78 | 17±2 | |
L3 | 432 | 23 | 1.47±0,67 | 23±2 |
C3 | 455 | 1.62±0,47 | 20±1 |
Table 1: Data obtained from spectroscopic titrations for the interaction of DNA with compounds L1-3 and C1-3.
Distribution coefficient (D)-An important physicochemical property that affects the biodistribution of a drug candidate is its lipophilicity. On the molecular level, it provides information about the inter- and intramolecular forces affecting drug transport through lipid structures. Thus, high lipophilicity facilitates the transport of drug through cell membranes [51]. More positive log P values correspond to more lipophilic complexes, whereas more negative log P values correspond to more hydrophilic ones. In addition, the cytotoxic activities of metal complexes against cisplatin-resistant human cancer cells strongly depend on their lipophilicity [52]. We found that all the compounds were lipophilic, namely L3 (1.32) > C3 (1.30) > C1 (1.29) > C2 = L2 (1.00) > L1 (0.82). Moreover, they are substantially more lipophilic than cisplatin (-2.21), carboplatin (-2.30) or oxaliplatin (-1.65), which have antitumor activity [52,53]. All the compounds showed lipophilicity that is in an optimal range (log P 0.5–3.5) for oral application which should allow sufficient absorption from the intestinal lumen into the bloodstream [54]. Previous studies on lipophilic anticancer complexes have shown that they may exert their toxicity by permeabilizing lipid membranes, resulting in disruption of the membrane structure thus exerting a cytotoxic effect [55-58].
Growth inhibition and cytotoxicity-The compounds were tested on six cell lines, representing tumors of different origins (prostate, breast, lung, cervix and colon), in addition to a murine breast tumor line, which are regularly use for in vivo testing of anticancer drugs. We used the SRB assay, which has the advantage over the more common tetrazolium assays as it distinguishes between a cytostatic effect, (reduction in cell proliferation) and a cytotoxic effect (decrease in the number of viable cells). Cisplatin and transplatin (0.1-30 µM) were included as control drugs. Table 2 shows that the compound C3 inhibited MCF7 cell growth at concentrations of 15.1 µM (GI50) and 28.5 µM (TGI), total growth inhibition of the PC3 cells below 17 µM and a cytotoxic effect on the latter cells at 28.7 µM. On the surface, it may appear that C3 shows a degree of “specificity” towards the PC3 prostate cell line. However, it must be remembered that anticancer drugs are generally only “specific” because they act primarily against dividing cells. Tumor cells divide faster than normal cells, and the PC3 cells show a particularly high rate of division in our hands, which may explain the results obtained. C1 and C2 showed no effect on the growth or viability of the cells, with the exception of a minor effect of C1 on the growth of the PC3 and HT29 cells (GI50=26.4 and 17.5 µM, respectively). Although lipophilicity and affinity for DNA, is an important factor in drug action, there are others, such as interactions with other molecules besides the target, solubility, transport within the cell and others which may account for the different activities of C1 and C3 against the cell lines, despite their similar P values and the higher affinity of C1 for DNA as measured in the test tube.
MCF7 | PC3 | A549 | HeLa | HT29 | 4T1 | |||||||||||||
Compounds | GI50 | TGI | LC50 | GI50 | TGI | LC50 | GI50 | TGI | LC50 | GI50 | TGI | LC50 | GI50 | TGI | LC50 | GI50 | TGI | LC50 |
C1 | >30 | >30 | >30 | 26.4 | >30 | >30 | >30 | >30 | >30 | >30 | >30 | >30 | 17.5 | >30 | >30 | >30 | >30 | >30 |
C2 | >30 | >30 | >30 | 24,3 | >30 | >30 | >30 | >30 | >30 | >30 | >30 | >30 | >30 | >30 | >30 | >30 | >30 | >30 |
C3 | 15.1 | 28.5 | >30 | 13.3 | 16.4 | 28.7 | >30 | >30 | >30 | >30 | >30 | >30 | >30 | >30 | >30 | >30 | >30 | >30 |
Cisplatin | 0.2 | >30 | >30 | 0.7 | 20.9 | >30 | 2.4 | 6.5 | 20.5 | 2.4 | 7.6 | 20 | 3.8 | 8.7 | 19.2 | 2.0 | 6.3 | 9.8 |
Transplatin | >30 | >30 | >30 | 0,20 | >30 | >30 | >30 | >30 | >30 | >30 | >30 | >30 | >30 | >30 | >30 | >30 | >30 | >30 |
Table 2: Cytostatic and cytotoxic effects of the compounds against six tumor cell lines. Cell viability was measured by the Sulphorhodamine B chromogenic assay after 48 hr incubation in the presence of the compounds. MCF7 human breast carcinoma, PC-3 - human prostate carcinoma, A459 - human lung carcinoma, HeLa - human cervical carcinoma, HT-29 - human colon carcinoma, 4T1 - murine breast carcinoma, GI50 - 50% growth inhibition, TGI - total growth inhibition, LC50 - 50% cytotoxicity. Concentrations are expressed in µM.
Phosphole derivatives (L1-3) and their gold(I) complexes (C1-3) were synthesized to evaluate their interaction with DNA and the main target of action of anti-cancer drugs. In general, we found that the relative viscosity of DNA for all complexes decrease probably due to bends or kinks in the DNA helix. All compounds showed weak interaction (non-covalent) with DNA, splitting the circular form of plasmid pBR322 and interacting weakly with the biomolecule. In addition, C1 and C2 have no effect on the growth or viability of the cells while C3 inhibited the cell growth of human breast carcinoma (MCF7) and human prostate carcinoma (PC3) cancer cells in vitro. Although lipophilicity and affinity for DNA are contributors to drug action, there are others, such as interactions with other molecules besides the target, solubility, transport within the cell and others which may account for the different activities of C1 and C3 against the cell lines, despite their similar P values and the higher affinity of C1 for DNA as measured in the test tube.
We gratefully acknowledge “Fondo Nacional de Ciencia Tecnología e Innovación de Venezuela” by financial support (Project No. PEI 2012-1054). We also thank Mercedes Fernández from Medicina Experimental, Instituto Venezolano de Investigaciones Científicas for his help with the electrophoresis studies.
Download Provisional PDF Here
Article Type: RESEARCH ARTICLE
Citation: Alfonso-Bueno S, Taylor P, Urdanibia I, Castro W, Otero Y (2018) Studies of the Interaction of Au(I)-Phosphole Complexes with DNA and their Relation to Biological Activity. J Med Chem Drug Des 1(1): dx.doi.org/10.16966/2578-9589.107
Copyright: © 2018 Alfonso-Bueno S, et al. This is an open-access article distributed under the terms of the Creative Commons Attribution License, which permits unrestricted use, distribution, and reproduction in any medium, provided the original author and source are credited.
Publication history:
All Sci Forschen Journals are Open Access