Figure 1: MTT assay with HOBs incubated in Mel-enriched media at 24 hr; *=significantly different from control and all other treatment groups; #=significantly different from control and HOBs incubated in 10 μM Mel; n=8; p<0.05 ANOVA.
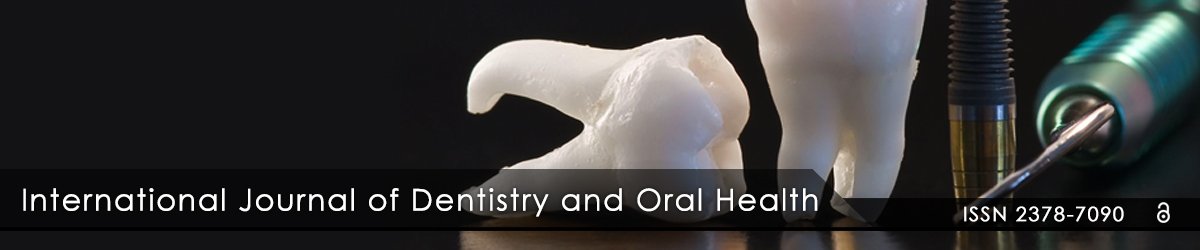
Full Text
AW Barone M Pringle D Nguyen R Dziak*
Department of Oral Biology, University at Buffalo School of Dental Medicine, Buffalo, NY, USA*Corresponding author: R Dziak, Department of Oral Biology, University at Buffalo School of Dental Medicine, Buffalo, NY, USA, Tel: 716 8293827; E-mail: rdziak@buffalo.edu
Introduction: Engineering biodegradable scaffolds for bone regeneration in osseous defects is an attractive alternative to harvesting grafts from living tissues. Calcium sulfate based scaffolds can be manufactured to have degradation profiles that are commensurate with new bone growth. However, concerns over the biomechanical strength of calcium sulfate have limited its use to filling small osseous defects. Nanoscale calcium sulfate exhibits enhanced physical properties and can successfully promote bone regeneration in critical-sized craniofacial bone defects. Previous studies show that this bio-ceramic can also accommodate sustainable growth factor delivery to bone cells in vitro. Recently, melatonin has received attention as a potential alternative to recombinant human proteins for use in enhancing the osteoinductive potential of synthetic bone scaffolds. Its clinical efficacy though may be limited by dose-related variation in its stimulatory effects on bone cells. To these ends, the incorporation of melatonin into nanoscale calcium sulfate may give rise to a novel scaffolding system the potential for clinical application in bone regenerative medicine.
Materials and Methods: Dose related osteogenic effects of melatonin on human osteoblastic cells were examined by measuring cellular viability and alkaline phosphatase activity. The bioactivity of melatonin released from nanoscale calcium sulfate was assessed through measurement of the viability of cells seeded with the scaffold. Scaffolds containing different amounts of melatonin were then fabricated and the release profiles from each group were characterized.
Results and Conclusion: Exposure of human osteoblastic cells to melatonin at micromolar concentrations increased cellular viability and alkaline phosphatase activity. Incorporation of melatonin into nanoscale calcium sulfate increased the viability of cells seeded with the scaffolds. Hence, melatonin released from the scaffolds retained its bioactivity. Release studies showed that nanoscale calcium sulfate can release melatonin in a sustained manner over time. The release profiles though were influenced by the amount of melatonin loaded. This study provided further evidence supportive of the potential use of nanoscale calcium sulfate as a tunable scaffold with the ability to deliver melatonin in quantities stimulatory to bone growth.
Nanoscale calcium sulfate; Bone regeneration; Osteoinduction; Melatonin; Controlled release; Human osteoblastic cells
nCS-Nanoscale Calcium Sulfate; CS-Calcium Sulfate; Mel-Melatonin; HOBs-Human Osteoblastic Cells; ALP-Alkaline Phosphatase
With over two million surgeries performed annually worldwide, bone grafting represents the most common method for augmenting osseous regeneration in a variety of medical fields that include dentistry, orthopedics, and neurosurgery [1]. Bone grafts are meant to serve as scaffolding structures supportive of new angiogenic and osseous in growths at wound sites. Clinically, surgeons still consider autografts to be the ‘gold standard’ for bone grafting procedures [2]. However, these grafting constructs are prone to causing various complications such as donor site morbidity, hematoma formation, and lengthened recovery periods [1-2]. Although the use of allografts has increased, these grafts pose risks for disease transfer and the triggering of adverse immune response. In hopes of circumventing some of the characteristic limitations associated with grafts harvested from living tissues, a vast number of FDA approved bone graft substitutes have emerged on the market in recent decades [1- 3]. Most of these synthetic constructs are hard, yet brittle, and lack intrinsic osteoinductive and osteogenic properties, are only osteoconductive [1].
An ideal synthetic scaffold for bone regeneration should be fully bio resorbable and possess a degradation rate commensurate with new bone formation at wound sites. Furthermore, the scaffold should be able to effectively stimulate the chemotaxis of bone precursor cells from the surrounding periosteum and promote their differentiation toward the osteoblastic phenotype [4]. To accomplish this, a popular strategy is to engineer osteogenic growth factors into the Three Dimensional (3D) architectures of synthetic scaffolding systems [5-8]. Growth factors bind to either cell surface or nuclear receptors. Such ligand-receptor binding modulates the activation and/or inhibition of signaling cascades that regulate protein production and gene transcription. Many of the osteogenic factors currently employed in bone tissue engineering are synthesized using recombinant DNA technology. To date, only three recombinant human osteogenic factors have received FDA approval for human use: rhBMP-2, rhBMP-7, and rhPDGF-BB [4]. However, their extent of clinical use is obfuscated by high production costs and limited commercial availability. They also have short biologic half-lives. For instance, the biologic half-life of rhPDGF-BB is ~2 min in vivo [9]. As a result of these drawbacks, some clinicians have transitioned to using Platelet-rich Plasma (PRP), which represents a more affordable and autologous source of osteogenic factors [10,11].
Currently, there is an emerging interest in the use of Melatonin (Mel)-a neuroendocrine hormone that is synthesized and secreted mainly by the pineal gland-as a therapeutic agent for improving bone regeneration in osseous defects [12-15]. In addition to the pineal gland, Mel synthesis also occurs in other areas of the body that include: the eyes, skin, intestines, gonads, and bone marrow [16-17]. Hence, its effects on the body are myriad and widely diverse. With respect to its therapeutic use in regenerative medicine, preclinical studies have shown that Mel can improve the quality of the bone fill in periodontal as well as craniofacial bone defects [14-15]. Furthermore, several in vitro studies indicate that Mel can enhance the proliferation and induce osteodifferentation in various human osteoblastic cell lines [18- 25]. Compared to recombinant human growth factors, Mel offers the following advantages: its low production cost allows for greater clinical accessibility, its small size makes it less susceptible to denaturation and its long biologic half-life (~60 min) allows for its clinical application to be less technique sensitive [13].
In addition to its proposed use in regenerative medicine, Mel has also received attention as a promising therapeutic adjuvant that can reduce the incidence of skeletal fracture and osteolysis in bone cancer patients [26]. However, while one in vitro study showed that exposure to millimolar Mel concentrations inhibits the proliferation of human osteosarcoma MG-63 cells, another in vitro study showed that exposure to millimolar Mel concentrations promotes apoptosis in preosteoblastic MC3T3 E1 cells [27-28]. As a result, systemic Mel administration in patients with bone tumors is prone to being confounded by having narrow therapeutic windows such that doses found to have optimal therapeutic effects in vitro may be too toxic to be considered safe in vivo.
Endogenous Mel is often present in the plasma at nanomolar concentrations [29]. Several in vitro studies have emphasized that exposure to Mel at micromolar concentrations can lead to increased viability in normal human osteoblastic cells (HOBs) [18- 24]. One possible way to optimize the therapeutic benefits of Mel on bone regeneration is by incorporating it into grafting materials accommodative of controlled drug release. However, while a vast number of bone scaffolding systems capable of sustainable drug delivery have been developed, only a few studies have considered loading these 3D constructs with Mel [30-33].
Nanoscale Calcium Sulfate (nCS) represents a novel scaffolding system with enhanced physical properties [34]. Compared to conventional sized medical grade Calcium Sulfate (CS), nCS has an exponentially increased surface area for cell attachment and osteogenic growth factor adsorption [34]. This feature confers nCS with increased loading capacity for growth factor content, thus increasing the bioavailability of the agent. It also allows it to accommodate controlled growth factor release [7,34]. In vitro studies have demonstrated that nCS scaffolds loaded with growth factors increase the viability of both HOBs and Mesenchymal Stem Cells (MSCs) [6-7,34]. Most recently, a preclinical study in canines revealed that nCS scaffolds can promote bone regeneration in critical sized mandibular defects [35].
The aim of the present to study was determine whether or not nCS scaffolds could accommodate the sustainable delivery of Mel by releasing the agent in amounts considered to be therapeutically relevant in vitro. Firstly, the specific range of Mel concentrations ideal for maximizing the viability and Alkaline Phosphatase (ALP) activity of human osteoblastic cells was established. This was done since the effects of Mel on bone cell viability and osteodifferentiation are reported to vary based on the concentration of agent used and the particular cell type examined [36]. Secondly, we assessed whether or not Mel released from nCS/Mel scaffolds remained biologically active. Lastly, we characterized the release kinetics of Mel from nCS/Mel scaffolds over time.
A series of Mel stock solutions were prepared by dissolving lyophilized melatonin powder (Sigma Aldrich, USA) in 5% ethanol (v/v) and PBS (Gibco, USA). All other chemicals and reagents used were purchased from Sigma-Aldrich unless stated otherwise.
Cells and cell culture
Primary HOBs were purchased from ScienCell Company (USA) and grown in 75 cm2 plastic culture flasks (Falcon, USA) with MEM-α (Gibco) supplemented with 10% Fetal Bovine Serum (FBS) and a 1% anti-mycotic solution. Media enriched with Mel was prepared by diluting aliquots from the stock solutions with appropriate volumes of MEM solvent. All materials used for cell culture maintenance were purchased from Gibco unless stated otherwise. Cultures were maintained at 37°C in a humidified incubator with 5% CO2. The media was refreshed every 2 to 3 days until cell confluence. Cells in passages 1 to 4 were used for the experiments.
MTT cell viability assay
The MTT [3 (4,5 dimethylthiazol 2 yl) 2,5 diphenyltetrazolium bromide] assay was conducted at 24 hr to examine the dose-dependent effects of Mel on HOB cellular viability. The concentrations of Mel chosen were based on data from previous reports [18,24]. HOBs incubated in MEM without Mel served as a control. Cells were seeded in 96 well plates (Costar, USA) and incubated in Mel poor or Melenriched media (10 μM, 50 μM, or 100 μM). At 24 hr, 13 μL of MTT reagent was added to each well, and the plate was incubated for another 4 hours. Then, 130 µL of Dimethyl Sulfoxide (DMSO) was added to each well, and the plate was incubated for a final 20 min. The Optical Density (OD) of each sample was measured by absorbance 540 nm in a microplate reader (Flex Station 3 Multi Mode Microplate Reader, USA).
ALP activity assay
The effect of Mel on the osteogenic differentiation of HOBs was evaluated at 48 with the ALP assay. Cells were seeded in 96-well plates (Costar) and incubated in Mel poor or Mel-enriched media (10 μM, 50 μM, or 100 μM). After a 48 hr incubation period, the media in each well was removed and replaced with 105 μL of 1% (v/v) Triton X-100 (Avantor Performance Materials, USA). The plate was then stored for 1 hr at 4°C. Afterwards, 50 μL of Amino-methyl Propanol (AMP) plus 50 μL of ρNpp reagent were added to each well, and the plate was incubated for a final 30 min. Cellular ALP levels were assessed by measuring the extent of enzymatic conversion of colorless ρnitrophenyl phosphate (ρNPP) to yellow ρnitrophenol (ρNp). The reaction was terminated by adding 50 μL of 0.5 N NaOH stop solution to each well. The OD of each sample was measured by absorbance at 405 nm in a microplate reader.
Preparation of nCS
Nanoscale calcium sulfate was prepared according to the procedure outlined by Park and colleagues [34]. Following the established protocol, 8.0 g of medical grade CS (CaSO4.2H2O; 99.9% pure) was dissolved in 4.0 L of distilled water. The homogeneous solution was then dispensed into small flasks that were frozen in liquid nitrogen at -196°C. Using a cryo-vacuum method, the dihydrate form of CS was converted into dihydrate nCS. The resultant white powder was then heated in a conventional oven at temperatures between 130 to 150°C to yield anhydrate nCS. Upon removal, the powdery product was sterilized via Glow-discharge Treatment (GDT). This served to produce a composition of nCS that was less susceptible to rehydration and more stable at room temperature.
Fabrication of nCS/Mel scaffolds
Scaffolds in the form of nCS/Mel discs were prepared as follows: 100 mg of nCS powder was mixed with 100 μL of PBS alone or with 100 μL aliquots containing different amounts of Mel. The resultant malleable white paste was then deposited in a polyvinyl siloxane mold and allowed to set for ~2.5 hr at room temperature. Once sufficiently hardened, the intact discs were removed from the mold and stored in sterilized glass containers at room temperature. Each disc was 5 mm wide and 3 mm thick and had a dry mass of 0.08 g.
Bioactivity study
Once a scaffolding system is demonstrated to be accommodative of sustainable drug delivery, it is necessary to determine whether or not the released agent remains biologically active. To accomplish this, the cellular viability of HOBs-incubated with nCS/Mel scaffolds at 37°C-was measured after 48 hr using the MTT assay. In this experiment, HOBs seeded with untreated nCS scaffolds served as a control. The treatment group consisted of HOBs seeded with nCS/ Mel scaffolds containing ~5.0 μg of Mel. At 48 hr, the MTT assay was conducted following the same protocol as mentioned before.
Release studies
A release study was conducted with nCS scaffolds mixed with Mel at different millimolar concentrations. The scaffolds in the treatment groups are denoted as follows: nCS/2.5 mM Mel, nCS/5 mM Mel, or nCS/10 mM Mel. A calibration curve (y=0.0011x+0.0045; R2=0.9987) was generated by measuring the optical densities of diluted Mel samples by absorbance at 254 nm. The wavelength for Mel absorption was chosen based on a previous report [37]. From the calibration curve, the amount of Mel released from the scaffolds was quantified at four predetermined time points (1,3,24, and 48 hr). For this experiment, nCS/Mel scaffolds (discs 5 mm wide and 3 mm thick with a dry mass of 0.08 g) were placed in separate wells of a 96 well plate and suspended in 200 μL of PBS. At each time point, the media was collected and replaced with an equal volume of PBS. The collected media from each sample was stored in a separate micro-centrifuge tube. At 48 hr, the amount of Mel present in each sample was quantified by absorbance at 254 nm in a microplate reader. Initial amounts loaded and cumulative amounts of Mel released from the nCS/Mel scaffolds are expressed in micrograms (μg). The amounts of Mel released were calculated as a percentage of the cumulative amount of Mel released at the specified time interval divided by the initial amount of Mel loaded into the nCS discs in μg.
Another release study was conducted with nCS scaffolds mixed with Mel at micromolar concentrations. For this experiment, the media was collected at only three predetermined time points (24,48, and 72 hr). There was a control and two treatment groups: nCS/10 μM-Mel and nCS/100 μM-Mel. At 72 hr, the cumulative amount of Mel released by each scaffold (disc 5 mm wide and 3 mm thick with a dry mass of 0.08) was quantified with an ELISA kit (Enzo Life Sciences, USA) and microplate reader. Initial amounts loaded and the cumulative amounts of Mel released from the nCS/Mel scaffolds are expressed in nanograms (ng). Because the detection range of the assay was between 0.08 50 ng/mL, the experimental samples were diluted accordingly as indicated by the manufacturer’s protocol. The Mel amounts released were calculated as a percentage of the cumulative amount of Mel released at the specified time interval multiplied by the corresponding dilution and divided by the initial amount of Mel loaded into the nCS discs in ng.
Statistical Analysis of Data
Results are presented as the means ± Standard Deviation (SD). All statistical computations were performed using Microsoft® Excel software (Microsoft Corporation, USA). All data were compared and evaluated using one-way Analysis of Variance (ANOVA). Statistical significance was accepted for p values ≤ 0.05.
Several authors report that Mel can enhance the bone-forming potential of osteoblasts both in vitro and in vivo [18-25,38-41]. However, the therapeutic effects of Mel on bone formation appear to be dose related. Multiple studies describe the osteogenic effects of Mel as being most robust at micromolar concentrations [18,21,24]. One in vitro study found the proliferative effects of Mel on Human Osteoblastic Cells (HOBs) to be maximal at doses between 50 and 100 μM [17]. Similarly, another in vitro investigation showed that Mel dose dependently increased the viability of HOBs cultured in media at concentrations between 50 and 200 μM [20]. Thus, it was hypothesized that exposure to Mel at micromolar concentrations would stimulate increases in the viability of HOBs. Treatment with Mel at 10 μM had the greatest stimulatory effect on cell viability (Figure 1). On the other hand, while HOBs treated with Mel at 50 μM and 100 μM showed increased viability relative to the control, they did not exhibit statistically greater viability relative to those treated with Mel at 10 μM. Our findings with are consistent other reports on dose related variation in the effects Mel on bone cell viability [18,21]. In our study, HOBs treated with Mel at higher concentrations (50 μM and 100 μM) may have already experienced the maximal proliferative effects of Mel and had become further differentiated. Such is the case as an attenuation of proliferation usually occurs when cells become further differentiated [42].
The osteoinductive potential of Mel was assessed by measuring the level of ALP activity exhibited by HOBs at 48 hr. HOBs incubated with Mel at 100 μM demonstrated the greatest level of ALP activity (Figure 2). However, HOBs treated with lower concentrations of Mel (10 μM and 50 μM) showed significantly less ALP activity relative to the control group. That said, others report observing dose-dependent increases in the ALP activity of HOBs at micromolar Mel concentrations in the range of ~10 to 200 μM [21]. Conversely, one in vitro study reports that the minimal Mel concentration required inducing osteodifferentiationas indicated by increases in ALP activity-in MSCs over a 2 day period is 50 nM [22]. In the same light, another in vitro study found that 50 nM was sufficient to induce osteodifferentiation-as indicated by increases in intracellular ALP mRNA transcripts-in preosteoblastic MC3T3 cells [19]. Our findings may be partly explained by differences in the types of Mel receptors expressed by HOBs.
Figure 2: ALP activity of HOBs incubated in Mel-enriched media at 48 hr; *=significantly different from control and all other treatment groups; #=significantly different from control and HOBs incubated in 10 μM Mel; n=8; p<0.05 ANOVA.
Bone cells express two different G-protein-coupled Transmembrane Mel receptors (MT1 and MT2) and one nuclear Mel receptor (RZR/ ROR) [43-45]. RZR/ROR expression might be regulated through Mel/ MT receptor binding. A previous study reports that MT2 receptor desensitization correlated with peak ALP activity in MSCs [20]. Evidence also suggests that aside from having lower ligand binding affinities compared to transmembrane Mel receptors, RZR/ROR receptors are likely to be implicated in signal transduction pathways that regulate the transcription of genes for bone marker proteins such as ALP [46,47]. In our study, it is possible that media enriched with Mel at 10 and 50 μM contained Mel in amounts sufficient to stimulate transmembrane Mel receptors yet not sufficient to effectively induce RZR/ROR mediated transcription of ALP over the 48 hr incubation period. This is supported by the relative magnitude of ALP expression by HOBs exposed to Mel at 100 μM versus Mel at lower concentrations. The increased ALP levels observed in the control versus HOBs exposed to Mel 10 and 50 μM can be attributed to the dose related and time dependent effects of Mel on osteodifferentiation.
In this paper, we have shown that Mel released from nCS retains its bioactivity at 48 hr. This was evidenced by the increased viability exhibited by HOBs seeded with nCS/Mel scaffolds versus HOBs seeded with untreated nCS scaffolds (Figure 3). This is noteworthy with respect to other studies conceiving the development of scaffolding systems supportive of localized and sustainable Mel delivery. Some of these require modifications of their surface chemistry in order to support Mel adsorption [30,33]. In theory, such molecular modifications could alter and/or compromise the bioactivity of released Mel. Hence, one of the advantages of using nCS in the fabrication of Mel loaded scaffolding systems is that modification of its surface chemistry is not required to support Mel adsorption.
Figure 3: Bioactivity evaluation with the MTT assay; Data represents relative viability of HOBs seeded on nCS or nCS/Mel scaffold; *=significantly different from control without Mel; n=8; p<0.05 ANOVA.
The results of the current study further demonstrate the potential for nCS to accommodate localized and sustainable growth factor delivery in vitro. This capability is important because it allows for Mel to be delivered specifically to cells responsible for regenerating osseous tissues in wound sites [48]. It is also important because it allows for more coordinated cell responses, such as the proliferation and differentiation of osteoblastic precursors. With respect to accommodating controlled release, nanomaterials possess numerous advantages over conventional-sized synthetic constructs [34,49]. In this paper, the release profiles of Mel from nCS/Mel scaffolds were evaluated in two separate experiments (Figures 4 and 5). In the first experiment, UV spectrophotometry was used to measure the amount of Mel released from nCS/Mel scaffolds with loadings between ~60 to 230 μg (Table 1). Scaffolds containing116 μg and 232 μg of Mel were characterized by release profiles bursts with initial (35% and 65%) followed by steadier release phases between 3 to 24 hr (Figure 4). By contrast, the released quantity of Mel from scaffolds containing 58 μg was less than 5% between 0 to 3 hr and less than 25% at 48 hr. Furthermore, these scaffolds displayed almost linear release kinetics throughout the entire experimental time period. In the second experiment, an ELISA kit was used to detect the amount of Mel released from nCS scaffolds with loadings between 230 and 2330 ng (Table 2). For both treatment groups, the quantity of Mel released was 1ess than 10% over 72 hr (Figure 5). Each group also exhibited sustained release profiles throughout the entire experimental time period. Similar to scaffolds loaded with 58 μg of Mel, a burst release did not occur in either of these treatment groups. Taken together, these findings suggest that nCS may serve as a tunable scaffold accommodative of the controlled delivery of Mel in quantities conducive to bone regeneration.
Figure 4: Cumulative amount of Mel released from nCS disc treated with Mel at millimolar (mM) concentrations; each data point represents the percentage amount of Mel (μg) released by nCS disc relative to the initial amount loaded; n=8.
Figure 5: Cumulative release of Mel from nCS disc treated with Mel at micromolar (μM) concentrations; each data point represents the percentage amount of Mel (ng) released by nCS disc relative to the initial amount loaded; n=8.
Released Melatonin (μg) | |||||
Scaffolds | 0 hr | 1 hr | 3 hr | 24 hr | 48 hr |
nCS/2.5 mM-Mel | 58.07 | 1.32 ± 0.13 | 2.13 ± 0.70 | 9.75 ± 1.59 | 12.92 ± 1.94 |
nCS/5 mM-Mel | 116.14 | 36.53 ± 2.72 | 41.94 ± 2.54 | 65.63 ± 4.56 | 67.73 ± 4.71 |
nCS/10 mM-Mel | 232.28 | 128.06 ± 11.56 | 142.30 ± 7.12 | 167.25 ± 12.48 | 167.40 ± 12.55 |
Table 1: First release experiment; Data collected using UV-spectrophotometry; Initial amount of Mel present in each nCS disc at 0 hr is expressed in micrograms/scaffold; Each other entry indicates the average cumulative amount of Mel released by the scaffolds at the specified time intervals.
Released Melatonin (ng) | ||||
Scaffolds | 0 hr | 24 hr | 48 hr | 72 hr |
nCS/10 μM-Mel | 232.28 | 3.72 ± 0.24 | 9.06 ± 0.64 | 14.63 ± 0.34 |
nCS/100 μM-Mel | 2322.80 | 60.39 ± 0.19 | 125.43 ± 0.75 | 185.82 ± 0.46 |
Table 2: Second release experiment; Data collected using an ELISA kit; Initial amount of melatonin present in each nCS disc at 0 hr is expressed in nanograms/scaffold; Each other entry indicates the average cumulative amount of Mel released by the scaffolds at the specific time.
Current reports on Mel loaded scaffolds mostly pertain to systems that utilize either β-tricalcium phosphate or variations of hydroxyapatite. A reported limitation to the use of calcium phosphate derivatives in synthetic bone grafting is the unpredictability that surrounds their biodegradation profiles [1-2]. With hydroxyapatite grafts, studies indicate that their relatively high calcium to phosphate ratios and crystalline structures prolong their resorption by phagocytic cells [50]. This leads to residual hydroxyapatite grafting material becoming embedded within the bone fill. The presence of such grafting remnants, in turn, compromises the intrinsic strength of the new bone by decreasing its resistance to fracture [2].
Compared to calcium phosphate based systems, grafts fabricated from CS are reported to be more bio-resorbable. In addition, they can be manufactured to have tunable degradation profiles that can closely match the rate of new bone formation [51]. However, reports on its weaker internal strength imply that its use should be limited to filling only small osseous defects [2,52]. Nanoscale calcium sulfate, on the other hand, has superior mechanical strength compared to CS and has been shown to successfully promote bone formation in critical sized craniofacial bone defects with favorable biodegradation such that even in large defects it appears to be completely resorbed and replaced by new bone [34-35]. The current study highlights the potential use for nCS as a bone grafting substitute and provides insightful suggestions on how to fabricate nCS/Mel scaffolds capable of releasing Mel in quantities that promote bone cell viability and osteodifferentiation.
In summary, this paper provided evidence supportive of doserelated variation in the effects of melatonin on bone cell viability and osteodifferentiation. Mel released from nCS/Mel scaffolds was shown to remain biologically active at 48 hr. Moreover, unlike other Mel loaded scaffolds; nCS did not require modification of its surface chemistry in order to support Mel adsorption. This paper also provided insight on the Mel loading capacity of nCS and its release kinetics. Collectively, our findings suggest that nCS may be a functional vehicle for the delivery of Mel in quantities capable of enhancing bone formation in osseous defects.
- Wang W, Yeung KWK (2017) Bone grafts and biomaterials substitutes for bone defect repair: A review. Bioact Mater 2: 224-247. [Ref.]
- Carson JS, Bostrom MP (2007) Synthetic bone scaffolds and fracture repair. Injury 38: S33-S37. [Ref.]
- Calori GM, Mazza E, Colombo M, Ripamonti C (2011) The use of bone-graft substitutes in large bone defects: any specific needs? Injury 42: S56-S63. [Ref.]
- Doll B, Aleef M, Hollinger JO (2008) Overview of fracture repair. In Musculoskeletal Tissue Regeneration (pp. 39-61). Humana Press.
- Xu L, Lv K, Zhang W, Zhang X, Jiang X, et al. (2012) The healing of critical-size calvarial bone defects in rat with rhPDGF-BB, BMSCs, and β-TCP scaffolds. J Mater Sci Mater Med 23: 1073-1084. [Ref.]
- He X, Dziak R, Mao K, Genco R, Swihart M, et al. (2013) Integration of a novel injectable nano calcium sulfate/alginate scaffold and BMP2 gene-modified mesenchymal stem cells for bone regeneration. Tissue Eng Part A 19: 508-518. [Ref.]
- Barone A, Morrell A, Dziak R (2016) Fabrication and Characterization of NanoCalcium Sulfate and Human Platelet Lysate as a Growth Factor Delivery System. Int J Dent Oral Health 2. [Ref.]
- Govender S, Csimma C, Genant HK, Valentin-Opran A, Amit Y (2002) Recombinant human bone morphogenetic protein-2 for treatment of open tibial fractures: a prospective, controlled, randomized study of four hundred and fifty patients. J Bone Joint Surg Am 84: 2123- 2134. [Ref.]
- Bowen-Pope DF, Malpass TW, Foster DM, Ross R (1984) Plateletderived growth factor in vivo: levels, activity, and rate of clearance. Blood 64: 458-469. [Ref.]
- Kanno T, Takahashi T, Tsujisawa T, Ariyoshi W, Nishihara T (2005) Platelet-rich plasma enhances human osteoblast-like cell proliferation and differentiation. J Oral Maxillofac Surg 63: 362-369. [Ref.]
- Anitua E (2001) The use of plasma-rich growth factors (PRGF) in oral surgery. Pract Proced Aesthet Dent 13: 487-493. [Ref.]
- Calvo-Guirado JL, Ramírez-Fernández MP, Gómez-Moreno G, Maté- Sánchez JE, Delgado-Ruiz R (2010) Melatonin stimulates the growth of new bone around implants in the tibia of rabbits. J Pineal Res 49: 356-363. [Ref.]
- Aravamudhan A, Ramos DM, Nip J, Subramanian A, James R, et al. (2013) Osteoinductive small molecules: growth factor alternatives for bone tissue engineering. Curr Pharm Des 19: 3420-3428. [Ref.]
- Maria S, Witt-Enderby PA (2014) Melatonin effects on bone: potential use for the prevention and treatment for osteopenia, osteoporosis, and periodontal disease and for use in bone-grafting procedures. J Pineal Res 56: 115-125. [Ref.]
- Najeeb S, Khurshid Z, Zohaib S, Zafar MS (2016) Therapeutic potential of melatonin in oral medicine and periodontology. Kaohsiung J Med Sci 32: 391-396. [Ref.]
- Conti A, Conconi S, Hertens E, Skwarlo-Sonta K, Markowska M (2000) Evidence for melatonin synthesis in mouse and human bone marrow cells. J Pineal Res 28: 193-202. [Ref.]
- Acuña-Castroviejo D, Escames G, Venegas C, Díaz-Casado ME, Lima- Cabello E, et al. (2014) Extrapineal melatonin: sources, regulation, and potential functions. Cell Mol Life Sci 71: 2997-3025. [Ref.]
- Nakade O, Koyama H, Ariji H, Yajima A, Kaku T (1999) Melatonin stimulates proliferation and type I collagen synthesis in human bone cells in vitro. J Pineal Res 27: 106-110. [Ref.]
- Roth JA, Kim BG, Lin WL, Cho MI (1999) Melatonin promotes osteoblast differentiation and bone formation. J Biol Chem 274: 22041-22047. [Ref.]
- Radio NM, Doctor JS, Witt-Enderby PA (2006) Melatonin enhances alkaline phosphatase activity in differentiating human adult mesenchymal stem cells grown in osteogenic medium via MT2 melatonin receptors and the MEK/ERK (1/2) signaling cascade. J Pineal Res 40: 332-342. [Ref.]
- Satomura K, Tobiume S, Tokuyama R, Yamasaki Y, Kudoh K, et al. (2007) Melatonin at pharmacological doses enhances human osteoblastic differentiation in vitro and promotes mouse cortical bone formation in vivo. J Pineal Res 42: 231-239. [Ref.]
- Sethi S, Radio NM, Kotlarczyk MP, Chen CT, Wei YH, et al. (2010) Determination of the minimal melatonin exposure required to induce osteoblast differentiation from human mesenchymal stem cells and these effects on downstream signaling pathways. J Pineal Res 49: 222-238. [Ref.]
- Son JH, Cho YC, Sung IY, Kim IR, Park BS, et al. (2014) Melatonin promotes osteoblast differentiation and mineralization of MC3T3-E1 cells under hypoxic conditions through activation of PKD/p38 pathways. J Pineal Res 57: 385-392. [Ref.]
- Altındal CD, James EN, Kaplan DL, Gümüşderelioğlu M (2019) Melatonin-induced osteogenesis with methanol-annealed silk materials. J Bioact Compat Polym 34: 291-305. [Ref.]
- Calvo-Guirado JL, Pérez-Albacete C, Sánchez CP, Boquete-Castro A, de Val JEMS, et al. (2015) Effects of melatonin on adult human mesenchymal stem cells in osteoblastic differentiation. An experimental in vitro study. Journal of Osseointegration 7: 23-32. [Ref.]
- Witt-Enderby PA, Radio NM, Doctor JS, Davis VL (2006) Therapeutic treatments potentially mediated by melatonin receptors: potential clinical uses in the prevention of osteoporosis, cancer and as an adjuvant therapy. J Pineal Res 41: 297-305. [Ref.]
- Liu L, Xu Y, Reiter RJ (2013) Melatonin inhibits the proliferation of human osteosarcoma cell line MG-63. Bone 55: 432-438. [Ref.]
- Qiu S, Tao ZB, Tao L, Zhu Y (2020) Melatonin induces mitochondrial apoptosis in osteoblasts by regulating the STIM1/cytosolic calcium elevation/ERK pathway. Life Sciences 248: 117455. [Ref.]
- Hevia D, Mayo JC, Quiros I, Gomez-Cordoves C, Sainz RM (2010) Monitoring intracellular melatonin levels in human prostate normal and cancer cells by HPLC. Anal Bioanal Chem 397: 1235-1244. [Ref.]
- Clafshenkel WP, Rutkowski JL, Palchesko RN, Romeo JD, McGowan KA, et al. (2012) A novel calcium aluminate-melatonin scaffold enhances bone regeneration within a calvarial defect. J Pineal Res 53: 206-218. [Ref.]
- Rahman MZ, Shigeishi H, Sasaki K, Ota A, Ohta K, et al. (2016) Combined effects of melatonin and FGF-2 on mouse preosteoblast behavior within interconnected porous hydroxyapatite ceramics-in vitro analysis. J Appl Oral Sci 24: 153-161. [Ref.]
- Yousef DA, Al Hessy AA, Abd Al Aziz AS, El Shamy ES (2018) Nanohydroxyapatite versus melatonin loaded on nanohydroxyapatite and nanohydroxyapatite with platelet rich fibrin on the treatment of intrabony defects. Tanta Dental Journal 15: 148-163. [Ref.]
- Miao Y, Chen Y, Liu X, Diao J, Zhao N, et al. (2019) Melatonin decorated 3D-printed beta-tricalcium phosphate scaffolds promoting bone regeneration in a rat calvarial defect model. J Mater Chem B 7: 3250- 3259. [Ref.]
- Park YB, Mohan K, Al-Sanousi A, Almaghrabi B, Genco RJ, et al. (2011) Synthesis and characterization of nanocrystalline calcium sulfate for use in osseous regeneration. Biomed Mater 6: 055007. [Ref.]
- Khobragade P, Jain A, Setlur SV, Andreana S, Dziak R, et al. (2015) Micro-computed tomography (CT) based assessment of dental regenerative therapy in the canine mandible model. SPIE Medical Imaging, International Society for Optics and Photonics 941710D-94171D. [Ref.]
- Sainz RM, Mayo JC, Rodriguez C, Tan DX, Lopez-Burillo S (2003) Melatonin and cell death: differential actions on apoptosis in normal and cancer cells. Cell Mol Life Sci 60: 1407-1426. [Ref.]
- Lai M, Jin Z, Tang Q, Lu M (2017) Sustained release of melatonin from TiO2 nanotubes for modulating osteogenic differentiation of mesenchymal stem cells in vitro. J Biomater Sci Polym Ed 28: 1651- 1664. [Ref.]
- Cutando A, Gómez-Moreno G, Arana C, Muñoz F, Lopez-Peña M, et al. (2008) Melatonin stimulates osteointegration of dental implants. J Pineal Res 45: 174-179. [Ref.]
- Muñoz F, López-Peña M, Miño N, Gómez-Moreno G, Guardia J, et al. (2012) Topical application of melatonin and growth hormone accelerates bone healing around dental implants in dogs. Clin Implant Dent Relat Res 14: 226-235. [Ref.]
- Calvo-Guirado JL, Gómez-Moreno G, Maté-Sánchez JE, López-Marí L, Delgado-Ruiz R, et al. (2015) New bone formation in bone defects after melatonin and porcine bone grafts: experimental study in rabbits. Clin Oral Implants Res 26: 399-406. [Ref.]
- Shino H, Hasuike A, Arai Y, Honda M, Isokawa K, et al. (2016) Melatonin enhances vertical bone augmentation in rat calvaria secluded spaces. Med Oral Patol Oral Cir Bucal 21: e122-e126. [Ref.]
- Meyer U, Meyer T, Schlegel W, Scholz H, Joos U (2001) Tissue differentiation and cytokine synthesis during strain-related bone formation in distraction osteogenesis. Br J Oral Maxillofac Surg 39: 22-29. [Ref.]
- Steinhilber D, Carlberg C (2005) Melatonin receptor ligands. Expert Opin Ther Pat 9: 281-290. [Ref.]
- Hardeland R, Pandi-Perumal SR, Cardinali DP (2006) Melatonin. Int J Biochem Cell Biol 38: 313-316. [Ref.]
- Reiter RJ, Tan DX, Manchester LC, Pilar Terron M, Flores LJ (2007) Medical implications of melatonin: receptor-mediated and receptorindependent actions. Adv Med Sci 52: 11-28. [Ref.]
- Mader S, Leroy P, Chen JY, Chambon P (1993) Multiple parameters control the selectivity of nuclear receptors for their response elements. Selectivity and promiscuity in response element recognition by retinoic acid receptors and retinoid X receptors. J Biol Chem 268: 591-600. [Ref.]
- Carlberg C (2000) Gene regulation by melatonin. Ann N Y Acad Sci 917: 387-396. [Ref.]
- Whitaker MJ, Quirk RA, Howdle SM, Shakesheff KM (2001) Growth factor release from tissue engineering scaffolds. J Pharm Pharmacol 53: 1427-1437. [Ref.]
- Okpala CC (2013) Nanocomposites-an overview. International Journal of Engineering Research and Development 8: 17-23. [Ref.]
- Wenisch S, Stahl JP, Horas U, Heiss C, Kilian O, et al. (2003) In vivo mechanisms of hydroxyapatite ceramic degradation by osteoclasts: fine structural microscopy. J Biomed Mater Res A 67: 713-718. [Ref.]
- Kuo ST, Wu HW, Tuan WH (2013) Resorbable calcium sulfates with tunable degradation rate. Journal of Asian Ceramic Societies 1: 102- 107. [Ref.]
- Beuerlein MJ, McKee MD (2010) Calcium sulfates: what is the evidence? J Orthop Trauma 24: S46-S51. [Ref.]
Download Provisional PDF Here
Article Type: RESEARCH ARTICLE
Citation: Barone AW, Pringle M, Nguyen D, Dziak R (2020) Dose-Related Effects of Melatonin on Human Osteoblastic Cells via in vitro Controlled Release from Nanoscale Calcium Sulfate. Int J Dent Oral Health 6(4): dx.doi.org/10.16966/2378-7090.325
Copyright: © 2020 Barone AW, et al. This is an open-access article distributed under the terms of the Creative Commons Attribution License, which permits unrestricted use, distribution, and reproduction in any medium, provided the original author and source are credited.
Publication history:
SCI FORSCHEN JOURNALS
All Sci Forschen Journals are Open Access