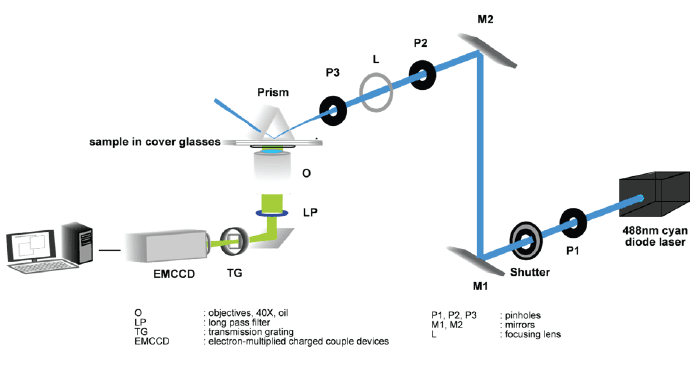
Figure 1: Schematic of TIRFM setup.
See-Lok Ho Hung-Wing Li Man Shing Wong*
Department of Chemistry, Hong Kong Baptist University, Hong Kong*Corresponding author: Hung-Wing Li and Man Shing Wong, Department of Chemistry, Hong Kong Baptist University, Hong Kong, E-mail: hwli@hkbu.edu.hk, mswong@hkbu.edu.hk
Fluorescence microscopy has been widely applied in many different biological aspects for sensing and imaging of biological samples, including cell imaging, real time monitoring of biomolecules and biomarkers detection. The commonly utilized light microscopy techniques are confocal, epi-fluorescence, multi-photon, total internal reflection fluorescence microscopy (TIRFM). Amongst all the fluorescence imaging techniques, TIRFM, also known as the evanescent field microscopy, has received considerable attention due to its unique properties. TIRFM is considered as a powerful technique, which enable in-vivo and in-vitro monitoring of dynamic and kinetic interaction of the biomolecules in single-molecules and single-cells level.
Total internal reflection occurs when the excitation light beam propagating through a dense medium (high refractive index) encounter another less dense medium (low refractive index) with an incident angle greater than the critical angle (θc ). The critical angle is derived by Snell’s law: θc = sin-1(n2 /n1 ), where n2 and n1 are the refractive index of the dense and less dense medium respectively. Instead of propagated through the less dense medium, certain amount of incident light is reflected off at the interface, this phenomenon is known as total internal reflection. At the same time, some of the incident energy will transmit through the interface and generated a standing electromagnetic field called evanescent field. Unlike other form of light, evanescence light decays in the intensity exponentially over a sub-wavelength distance. The intensity of the evanescent field (Iz ) at any position (z) can be calculated by Iz =I0 exp-z/d, where I0 is the intensity at z=0, d is the penetration depth of the evanescent field that can be obtained by d=λ / (4π (n12 sin-1θ - n22 )1/2), where λ is the wavelength of the excitation light beam in vacuum. The penetration depth of the light beam is typically ~100-300 nm. In a prism-type TIRFM the penetration depth can be controlled by three factors: (i) the wavelength of the excitation source, the longer the wavelength of the light source, the deeper the evanescent field is. (ii) The incident angle of the light source, the penetration depth decreases while increasing the incident angle. The last but not least, (iii) refractive index of the less dense medium, as the refractive index of the less dense medium increases, the penetration depth also increases.
In TIRFM since the intensity of the evanescent field decays exponentially, fluorophores closer will be excited more strongly than the fluorophores away from the interface, which gives a high-contrast image of the fluorophores near the interface. In addition, because of the penetration depth of the evanescent field is very shallow, only fluorophores within the field will be excited, while the rest in the bulk solution will remain “silent”. It gives a high signal-to-noise ratio and reduces the photo-damage on the analytes.
Figure 1: Schematic of TIRFM setup.
Reports have demonstrated the capability of TIRFM for in-vitro quantification of disease associated biomarkers for instances, a singlemolecule detection assay for quantification of microRNAs (miRNAs) and circulating miRNAs in cell lysate and human serum samples. The developed assays are highly sensitive, specific, and successively differentiate different stages of cancer [1,2]. Researchers also applied TIRFM imaging system to monitor the aggregation kinetics of beta-amyloid peptide [3-10]. Simon group reported the utility of TIRFM as one of the imaging approaches to reveal a previously unobserved view of the genesis of individual virions. They were also able to explore the different parameters of viral assembly that are inaccessible with other conventional techniques [11]. Nosrati et al. [12] monitored the sperm motion which is well-known to be a crucial role in fertilization and were able to resolve the nature of this motion. Using TIRFM, they selectively imaged motile human and bull sperm to revealing a two-dimensional (2D) ‘slither’ swimming mode. This behavior was found to be distinguishable from bulk and near-wall swimming modes. The influence of media viscosity was studied and a strategy was suggested for the human sperm that is suitable for the highly viscous and confined lumen within the fallopian tube [12]. Real-time monitoring and tracking of single molecules or single cells in wide field mode is very attractive for high-throughput studies. Coupling several lasers of different excitation wavelengths which can be switched in microseconds precisely with an acousto-optical tunable filter (AOTF) allows sophisticated multicolor biomolecular imaging and tracking [13]..1
TIRFM is no longer limited to 2D imaging; Fang group demonstrated that the z-positions of fluorescent nano particles close to the cell baso lateral membrane can be extracted by collecting the fluorescence intensities at different incident angles. Once the incident angle is reduced to be in the sub-critical range, the TIRFM works as a pseudo-TIRFM by which the whole cell-body can be monitored from bottom to top [14]. Three-dimensional tracking strategies are hence established [13].
In short, highly sensitive single-molecules and single-cells fluorescence microscopy techniques, like TIRFM, are powerful tools for addressing clinical and scientific challenges. However, novel with high spatial resolution microscopy techniques are valuable tools to evaluate the functionality of those interested biomolecules in a complex cellular environment.
Download Provisional PDF Here
Aritcle Type: Opinion Article
Citation: Ho LS, Li WH, Wong MS (2016) Seeing Molecules Interact with Total Internal Reflection Fluorescence Microscopy. J Biochem Analyt Stud 1(1): doi http://dx.doi.org/10.16966/2576-5833.102
Copyright: © 2016 Ho LS, et al. This is an open-access article distributed under the terms of the Creative Commons Attribution License, which permits unrestricted use, distribution, and reproduction in any medium, provided the original author and source are credited.
Publication history:
All Sci Forschen Journals are Open Access